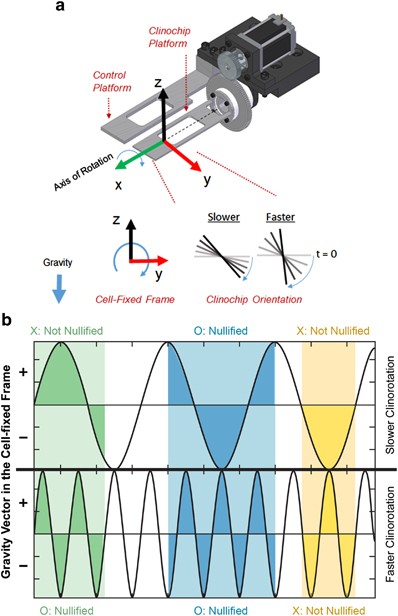
Effects of angular frequency during clinorotation on mesenchymal stem cell morphology and migration
- Select a language for the TTS:
- UK English Female
- UK English Male
- US English Female
- US English Male
- Australian Female
- Australian Male
- Language selected: (auto detect) - EN
Play all audios:

ABSTRACT AIMS: To determine the short-term effects of simulated microgravity on mesenchymal stem cell behaviors—as a function of clinorotation speed—using time-lapse microscopy. BACKGROUND:
Ground-based microgravity simulation can reproduce the apparent effects of weightlessness in spaceflight using clinostats that continuously reorient the gravity vector on a specimen,
creating a time-averaged nullification of gravity. In this work, we investigated the effects of clinorotation speed on the morphology, cytoarchitecture, and migration behavior of human
mesenchymal stem cells (hMSCs). METHODS: We compared cell responses at clinorotation speeds of 0, 30, 60, and 75 rpm over 8 h in a recently developed lab-on-chip-based clinostat system.
Time-lapse light microscopy was used to visualize changes in cell morphology during and after cessation of clinorotation. Cytoarchitecture was assessed by actin and vinculin staining, and
chemotaxis was examined using time-lapse light microscopy of cells in NGF (100 ng/ml) gradients. RESULTS: Among clinorotated groups, cell area distributions indicated a greater inhibition of
cell spreading with higher angular frequency (_P_<0.005), though average cell area at 30 rpm after 8 h became statistically similar to control (_P_=0.794). Cells at 75 rpm clinorotation
remained viable and were able to re-spread after clinorotation. In chemotaxis chambers, clinorotation did not alter migration patterns in elongated cells, but most clinorotated cells
exhibited cell retraction, which strongly compromised motility. CONCLUSIONS: These results indicate that hMSCs respond to clinorotation by adopting more rounded, less-spread morphologies.
The angular frequency-dependence suggests that a cell’s ability to sense the changing gravity vector is governed by the rate of perturbation. For migration studies, cells cultured in
clinorotated chemotaxis chambers were generally less motile and exhibited retraction instead of migration. SIMILAR CONTENT BEING VIEWED BY OTHERS CELLULAR MECHANOTRANSDUCTION OF HUMAN
OSTEOBLASTS IN MICROGRAVITY Article Open access 21 March 2024 IMPAIRMENT OF 7F2 OSTEOBLAST FUNCTION BY SIMULATED PARTIAL GRAVITY IN A RANDOM POSITIONING MACHINE Article Open access 07 June
2022 SIMULATED LUNAR MICROGRAVITY TRANSIENTLY ARRESTS GROWTH AND INDUCES OSTEOCYTE-CHONDROCYTE LINEAGE DIFFERENTIATION IN HUMAN WHARTON’S JELLY STEM CELLS Article Open access 04 May 2024
INTRODUCTION Intricate multi-scale interactions among cells, tissues, and organs fundamentally govern human health, which has evolved on Earth under a constant gravitational load of 1 g (9.8
m/s2). The biological mechanisms underlying the role of gravity in human health remain poorly understood, but their elucidation is necessary for enabling long-term manned space exploration.
Numerous studies, supported by the National Aeronautics and Space Administration and other space agencies, have shown deleterious effects of space travel on the human body, such as
accelerated bone loss,1,2 muscle tissue degeneration,3 and others.4 Importantly, these observations may have broader implications beyond spaceflight applications to provide a more detailed
understanding of diseases on Earth. In particular, the musculoskeletal system has historically been a focal point in space biology research, because of the strikingly adverse changes that
occur in astronauts.5 More recently, studies have begun to investigate mesenchymal stem cells for their roles in musculoskeletal lineage determination,6 bone repair,7 and tissue
maintenance.8 Although the most relevant environment for performing microgravity research is in space, competition to use space-based facilities, like the International Space Station, is
fierce and is further complicated by substantial time and resource investments.9 As a result, lower-cost and logistically simpler alternatives are attractive and include sounding rockets10
and parabolic flights,11 or in-lab devices such as random positioning machines12 and clinorotation devices (clinostats)13 to simulate microgravity. The selection of a specific technique
usually depends on accessibility, cost, experimental design, and research question. Clinostats are among the most accessible methods to simulate microgravity, and they allow researchers to
study living cells using standard laboratory tissue culture supplies.14 Clinorotation experiments have provided significant insight into the behavior of biological organisms in space. The
technique was originally developed to study gravitropism in plant development, and has revealed that gravity serves a key role in statocyte function, which is believed to be directly
involved in plant gravisensing and growth patterns.15 In animal and human cells, clinorotation has been used to recreate experiments that would otherwise be challenging to perform in space,
such as high-resolution microscopy16 and assessment of stem cell differentiation.17 Cellular changes in microgravity have been associated with disruptions in the cytoskeleton and,
consequently, changes in cell morphology and behavior.18,19 Although clinorotation is a standard ground-based tool, previously reported studies on the effects of simulated microgravity in
mesenchymal stem cells have yielded conflicting findings (Table 1). For instance, some researchers have shown that microgravity enhances proliferation,17 whereas others have demonstrated
inhibition.20 Chondrogenic differentiation has also been found to be either promoted21 or suppressed.22 Similar inconsistencies have also been highlighted in a recent review.23 Some of these
observed discrepancies could be due to differences in culture media formulation and cell source, but it is also possible that the choice of clinorotation parameters used for an experiment
may substantially influence cell behavior. A two-dimensional clinostat rotates a sample along the longitudinal axis to produce a time-averaged nullification of the gravity vector.
Theoretically speaking, to simulate microgravity effectively, the period of rotation should be shorter than some time constant that governs the rate processes involved in the cellular
gravisensing machinery that enable a cell to ‘perceive’ and respond to the changing trajectory of the gravity vector. For conventional clinostats, however, there are also practical
considerations that limit the angular frequency of rotation.24 Specifically, rotation speed must be adjusted to balance sedimentation forces, centrifugal and Coriolis effects, and Stokes’
drag. Because of these constraints, the parameters of angular rotation depend on the particular design and implementation of each experimental system. To minimize the effects of these
extrinsic stimuli, we recently developed a lab-on-chip clinorotation device (clinochip, Figure 1a) that confines adhered cells within a small region along the axis of rotation, limiting
residual accelerations to levels below 10−4g at different rotation speeds.25 Moreover, the clinochip is amenable to time-lapse microscopy, which enables us to characterize the kinetics of
cell spreading, changes in morphology, and migration with minimal disruption to the simulated microgravity environment. In this study, we used this device to investigate how angular
frequency affects human mesenchymal stem cell (hMSC) behavior. On the basis of the concept that there are specific rate processes that govern cellular gravisensing (Figure 1b), we
hypothesize that hMSCs would exhibit angular frequency-dependent responses. The results of this work demonstrate that hMSCs can in fact ‘detect’ differences in rotation speed in a manner
that causes heterogeneous population shifts toward more rounded morphologies and retraction of cell area. This could have significant implications on our basic understanding of stem cell
regulation as well as on strategies that are being used in stem cell-based applications. MATERIALS AND METHODS MATERIALS hMSCs were obtained from a commercial source (PT-2501, Lonza,
Walkersville, MD, USA), and confirmed to be mycoplasma-free. Clinochip fabrication required microscope slides (12-544-1, Fisher Scientific, Waltham, MA, USA) and 0.25- mm-thick
polydimethylsiloxane sheets (HT-6240, Rogers Corporation, Rogers, CT, USA). Fluorescence visualization was performed for actin using 5% (w/v) Texas red phalloidin (Life Technologies,
Gaithersburg, MD, USA) and for focal adhesions using 1 μg/ml fluorescein-conjugated anti-vinculin (MA1–34629, Life Technologies). Fibronectin (354008, BD Biosciences, San Jose, CA, USA) was
used to enable cell adhesion, and nerve growth factor (NGF; 7S-NGF, Life Technologies) was used for migration experiments. Calcein-AM and ethidium homodimer-1 (L-3224, Life Technologies)
were used to perform cell viability assays. EXPERIMENTAL SETUP In general, we used a single experimental system for all of our experiments, as detailed previously.25 In brief, the system
consists of a custom microscope-mounted gear system, driven by a computer-controlled stepper motor that rotates a small slide holder.25 This slide holder can accommodate various custom and
commercially available lab-on-chip devices. A non-rotating slide holder enables having a static (standard gravity) control condition performed concurrently. The entire system is enclosed in
an environmental chamber (Precision Plastics, Beltsville, MD, USA) and installed on an Olympus IX-81 epi-fluorescence microscope (Center Valley, PA, USA). CELL MORPHOLOGY PLATFORM A custom
‘clinochip’ device was used for visualizing cell spreading (that is, changes in cell morphology over time), and fabricated according to our previously described protocol.25 This clinochip
consists of three layers comprising polydimethylsiloxane sandwiched between two layers of glass. In brief, we used a high-resolution razor cutter (FC8000, Graphtec, Irvine, CA, USA) to trim
stock polydimethylsiloxane sheets. The sheet was cut to length and width dimensions of microscope slides and with a single 1-mm wide, 30-mm long channel at the center. A second microscope
slide was used as the third layer. Prior to assembly, two 1-mm diameter holes had been made in the top slide using a sandblaster (6500, Airbrasive, Piscataway, NJ, USA) to provide access to
the channels. The three layers were energetically bonded using a high-frequency corona treater (BD-20AC, Electrotechnic Products, Chicago, IL, USA). The clinochip was first sterilized for 20
min in a UV chamber. To promote cell attachment, the channel was then treated with 100 μg/ml fibronectin for 1 h and washed with phosphate-buffered saline before use. Passage 3 (P3) and P4
hMSCs were seeded into clinochip channels and incubated at 37 °C for 10 min to allow for cell attachment, followed by commencement of clinorotation. CELL MIGRATION PLATFORM Cell migration
was analyzed using commercially available Ibidi Chemotaxis slides (μ-Slide Chemotaxis 2D, Ibidi, Munich, Germany). The slides fit perfectly in our clinorotation device and the 2×1 mm
dimensions of the chamber are suitable for simulated microgravity, as they constrain cells to a narrow region about the axis of rotation to minimize centrifugal effects. First, a 100 μg/ml
fibronectin solution was injected into the viewing chamber and incubated for 1 h, followed by three phosphate-buffered saline washes. Then, P3 and P4 hMSCs were plated and incubated at 37 °C
for 12 h of initial seeding time. Our pilot experiments showed that after 12 h the spreading areas of hMSCs reach a plateau so that cell spreading would not confound the cell migration
data. As a first step, we chose to study hMSC chemotaxis mediated by NGF, one of several growth factors identified in this active field of research.26 Mesenchymal stem cells have been shown
to express receptors to neurotrophic factors including NGF,27 and also secrete NGF during tissue repair.28 In these migration experiments, NGF was prepared as a 100 ng/ml solution and
injected into the top ports of the chamber. Following manufacturer’s instructions, the same volume was aspirated from the opposite port to create a chemical gradient. CLINOROTATION AND
TIME-LAPSE IMAGING Both morphology and chemotaxis platforms were rotated along the axis of the channels in which cells were cultured. Residual accelerations are one to two orders of
magnitude smaller than conventional clinostats, even at 75 rpm.25 Before the experiment, we made sure that the channels did not contain any bubbles, to minimize any other external effects
such as shear stress and dehydration. During imaging, we constrained our analyses to cells near the axis of rotation, away from the sides of the channel, to avoid potential edge effects. At
the appropriate times (10 min after seeding for morphology assays; 12 h after seeding for chemotaxis and migration assays to allow the cells to become fully spread), clinorotation was
initiated at 30, 60, or 75 rpm and maintained over the course of 8 h. Clinochips at 0 rpm (non-rotated) were used as a control (standard gravity). During each hour, rotation was paused for a
total of 60 s, and a set of bright-field images of the clinochip were acquired at ×200 magnification. At the end of the 8 h period, cells were fixed in 4% paraformaldehyde, and
fluorescently stained for actin and vinculin. Captured images were analyzed using ImageJ (National Institutes of Health). CELL VIABILITY AND RECOVERY AFTER CLINOROTATION Cell viability was
determined using calcein-AM (green) to indicate intracellular esterase activity for live cells and ethidium homodimer-1 (red) to indicate loss of plasma membrane integrity for dead cells.
Calcein (final concentration 4 μM) and ethidium homodimer (final concentration 2 μM) were added to cell culture media that was used to plate hMSCs in fibronectin-treated clinochips. After 10
min of attachment, clinochips were either rotated at 75 rpm or maintained at standard gravity conditions (non-rotated control) and fluorescence micrographs were taken at 1, 4, and 8 h
during clinorotation. In addition to the live/dead assays, we acquired light micrographs of cell morphology in standard gravity following exposure to (a) 1 or 4 h of 30 rpm clinorotation,
(b) 1 or 4 h of 60 rpm clinorotation, or (c) 1, 4, or 8 h of 75 rpm clinorotation. After termination of the clinorotation treatment, images were acquired in standard gravity every 30 min for
4 h, and then analyzed for cell area and shape factor using ImageJ (National Institutes of Health). STATISTICAL ANALYSES Because no prior publications have quantified cell areas during
clinorotation, we relied on pilot data for descriptive statistics to make sample size calculations. Assuming a critical significance level of _α_=0.05, statistical power of 0.9 (_β_=0.1),
and a detectable difference equal to the population s.d., we performed a power analysis to calculate an estimated sample size of _n_=23, using the method of Sokal and Rohlf.29 To ensure
adequate statistical power for cell area measurements, we chose to include at least _n_=30 for each sample group (Table 2), acquired over several experimental replicates. All data are
expressed as mean±s.e.m. Variance did not markedly differ between groups (less than fourfold). Average cell areas among different clinorotation speeds were first log transformed to improve
normality. Differences between clinorotation groups were then statistically analyzed using the Welch’s _t_-test for samples with unequal variance and unequal sample size, with Tukey _post
hoc_ tests for multiple pairwise comparisons. Using Welch's _t_-test for samples with unequal variance and sample size, we analyzed differences in cell velocity and directionality
between 0 (_n_=15) and 75 rpm (_n_=6) only for cells that were actively migrating. Similarly, we used Welch's _t_-test with Tukey post hoc test for multiple pairwise comparisons to
analyze differences in cell area between 30 and 75 rpm (at 0, 1, 2, 3, and 4 h) only for cells that were actively retracting (_n_=28). RESULTS SIMULATED MICROGRAVITY AFFECTS CELL SPREADING
Simulated microgravity had a profound effect on both the extent and kinetics of cell spreading (Figure 2). After the initial 10-min cell attachment and before rotation commenced, all of the
groups possessed similar cell areas of ~500 μm2. We found that hMSCs in the non-rotated 0 rpm condition (i.e., standard gravity) markedly increased spreading areas within 1 h after plating,
reaching an average of 2000 μm2. Their spreading area increased gradually with time, until they reached an average of 3000 μm2 by 8 h. For clinorotated groups, cell spreading was impeded
almost immediately. At 1 h, cells in the 30 rpm group were significantly smaller than control with an average cell area slightly above 1000 μm2; cells at 60 and 75 rpm were both
significantly different from 0 and 30 rpm, remaining rounded and small. The effects of clinorotation continued to affect cell spreading over the course of the entire 8 h experiment in a
rotation speed-dependent manner. By 8 h, we measured a statistically similar but smaller average area of 2750 μm2 for cells at 30 rpm. In contrast, the 60 rpm group had an average area of
2192 μm2, and the 75 rpm group had the lowest cell area of 1500 μm2, both statistically smaller than 0 and 30 rpm. From a kinetics perspective, the time required for cell spreading areas to
reach 2000 μm2 was within 1 h for non-rotating controls, within 4 h for 30 rpm, and within 8 h for 60 rpm. Our experiments did not run long enough to determine whether or not cells would
reach this mark for the 75 rpm condition, but based on the observed trends, it appears that cell spreading no longer increases after 7 h. These results indicate that cell spreading is
markedly affected by angular frequency and that these effects can be observed as soon as 1 h after clinorotation starts. Finer temporal resolutions and longer durations in future experiments
may provide more detailed insight on the time constant of important cellular processes associated with cellular gravisensing. One notable observation we made was that cells within each
clinorotation speed were not uniformly spread. As individual cells did not accurately represent the population characteristics, we plotted the group-wise distributions of cell morphologies
at various time points (Supplementary Figure 1). The 0 rpm control group adopts a broad distribution of cell areas within the first hour, followed by the 30 and 60 rpm groups. By 8 h, it is
clear that the 0, 30, and 60 rpm cell populations encompassed comparable ranges of cell areas. The 75 rpm group, however, exhibited a much more narrow distribution. Likewise, when staining
for filamentous actin we found that cells also spanned a range of cell shapes within each group (Supplementary Figure 2). There were no discernable differences in actin organization between
cells of similar morphology (elongated or rounded), even when comparing across different clinorotation conditions. This suggests that the observed population differences with angular
frequency is caused by disparate effects on individual cells, perhaps by triggering/overcoming some intrinsic signal within cells to reduce cell adhesion, suppress actin stress fiber
formation, and consequently induce cell rounding. It is unclear whether simulated microgravity directly affects actin stress fiber assembly to result in cell rounding, or whether cell
rounding induced by simulated microgravity is what inhibits stress fiber formation. To quantify these differences in cell shape as a function of clinorotation speed, we computed circularity
(circularity=4_π_*area/perimeter2), as it accommodates more abstract shapes than an aspect ratio calculation. We assigned circularity values above 0.6 to cells that were considered rounded
(Figure 3, black), values between 0.3 and 0.6 to semi-elongated cells (Figure 3, hatch) and values below 0.3 to elongated cells (Figure 3, gray). As expected from the previous area
measurements, Figure 3 clearly shows that increasing clinorotation speed results in a larger percentage of rounded cells in its population. The number of elongated cells was significantly
reduced with increasing clinorotation speed, and no elongated cells were found at 75 rpm. Plotting cell circularity against cell area for each clinorotation speed at different time points,
we clearly see a temporal increase in area associated with cell elongation in 0 rpm control cells (Supplementary Figure 3). This trend becomes disrupted with exposure to 30 and 60 rpm
clinorotation, as cells tended to exhibit a broad range of circularity and area. However, only at 75 rpm the does cell population maintain high circularity and smaller areas across time
points (1, 4, and 8 h). Overall, our morphology analysis demonstrates that the cell population at 75 rpm possesses a more consistent morphology (average area, cell distribution, circularity
versus area over time) over time. CELL VIABILITY AND RECOVERY AFTER CLINOROTATION To determine whether there was any loss of viability or if rounded cells were undergoing apoptosis, we
exposed cells to 75 rpm and used a live/dead assay after 1, 4, and 8 h of clinorotation (Figure 4b). We found no difference in cell viability or changes in cell number when compared with
control; in fact, the majority of cells were alive (>90%). Under light microscopy, we observed no evidence of apoptosis (i.e., membrane blebbing) for the cells that were not viable. This
also indicates that our clinochip system can be used effectively without loss of cells due to lifting or cell death for at least 8 h. We investigated whether clinorotation effects on cell
morphology are reversible by performing time-lapse microscopy after exposure to 1, 4, and 8 h of 75 rpm clinorotation. Following cessation of clinorotation, all cells increased their areas
and round cells became more elongated, similar to most of the cells in standard gravity controls (Figure 4a). Similar experiments were also repeated for 30 and 60 rpm after 1 and 4 h of
clinorotation (Supplementary Figure 4). SIMULATED MICROGRAVITY INHIBITS CELL MIGRATION BY INDUCING CELL ROUNDING For cell migration experiments, cells were seeded in Ibidi chemotaxis chips
with a chemical gradient of 100 ng/ml NGF to promote cell migration. Under clinorotation, only a few cells migrated, whereas most cells did not (see Table 2 for cell numbers). Cells that
were actively migrating at 75 rpm had a similar velocity (_P_=0.391) and directionality (_P_=0.822) when compared with control cells (Figure 5). Cells that were not migrating under simulated
microgravity exhibited morphological retraction (Figure 5). In other words, cell areas changed from fully spread morphologies to more rounded ones. This phenomenon is in agreement with our
observations in cell-spreading experiments, even when the initial conditions were different (10 min for spreading assays versus 12 h for chemotaxis and migration assays). We found that
during clinorotation cells retracted their area, but did so independently of rotation speed (‘0 h’ _P_=0.539, ‘1 h’ _P_=0.288, ‘2 h’ _P_=0.963, ‘3 h’ _P_=0.848, ‘4 h’ _P_=0.689, between 30
and 75 rpm). After 4 h of clinorotation during chemotaxis, cells at 30 and 75 rpm reached an average area of 2500 μm2, which is a value similar to the spreading experiments for 30 rpm at 8 h
(_P_=0.962 between 30 rpm, _P_=0.975 between 75 rpm). However, we did not conduct experiments long enough to determine steady state–retracted cell areas. Our results indicate that the
cellular response to simulated microgravity is more dominant than chemotactic signals, suggesting the role of migration of hMSCs to tissue repair sites might be suppressed in microgravity.
We base this conclusion on our observations of response to NGF at a concentration of 100 ng/ml. However, these results were obtained with only one concentration of NGF and it is possible
that different concentrations or other chemotactic molecules can elicit a stronger chemotactic response. For example stromal-derived factor-1, which is known to be a strong homing signal for
hMSCs.30 Nevertheless, because the response of adhered cells to clinorotation is retraction of cellular processes, we believe that the behavior will be similar, unless the chemotactic agent
can also enhance cell adhesion. DISCUSSION Understanding how angular frequency, or rotation speed, of clinorotation affects cell behavior is a critical aspect of microgravity simulation
experiments, so that the role of the changing gravity vector in cellular regulation can be appropriately considered. In this work we show that hMSCs can behave differently depending on
clinorotation speed. In particular, although average cell area increased more slowly at 30 rpm compared with non-rotated controls, it continues along an upward trajectory at 8 h and seems to
be converging with control values. For higher rotational speeds, cell area increases even more slowly, and appears to reach a plateau by 8 h at 75 rpm. This suggests that changes in cell
morphology may approach a limit at an angular frequency that is close to 75 rpm, and almost certainly must exceed 30 rpm. Although these results are relevant to our clinochip configuration,
results may vary for other clinostat devices, particularly for those that support cells in suspension. Our results are consistent with other research showing that rotation speed affects
animal cells, plants, and bacteria (_Escherichia coli_). For example, a study on osteoblastic ROS 17/2.8 cells reported that rotations at 10 and 40 rpm did not exhibit reproducible,
detectable changes from stationary control cells.31 Only a speed of 50 rpm showed reproducible changes in actin cytoskeleton and cell surface integrin β1 and apoptosis. The same effect has
been observed with E. coli,32 which exhibited differential response as a function of clinorotation speeds from 10 to 50 rpm. Interestingly, the difference between 40 and 50 rpm is only
0.1674 of a cycle per second, indicating that cells can be highly sensitive to small changes in angular frequency. Although a precise gravisensing mechanism has yet to be determined, it must
be one that can detect subtle alterations to external forces in a short period of time. Our data indicate that it is linked to pathways that are involved in cell spreading and cytoskeletal
organization, two phenomena that have been observed for different cell types. Potential candidates could be one or more molecules already known to play important roles in cellular
mechanoregulation, such as the Rho family of GTPases,33 ion mechanosensitive channels,34 intracellular calcium,35 nuclear deformations,36 focal adhesions, or other cell surface receptors.37
Although mechanosensitive pathways seem the most direct apparatus for gravisensing, others have also suggested the importance of external environmental factors including fluid shear, fluid
and nutrient exchange, oxygen content, buoyancy, and changes in the extracellular matrix.38 Thus, clinostats used to probe candidate gravisensing receptors should minimize external
environmental variables that arise from clinostat operation. In the context of a proposed framework for cellular gravisensing, the current results are consistent with our working
hypothetical model (Figure 1b). Standard gravity would constitute a constant gravitational stimulus and be detectable to all cells. With intermediate timescales of gravitational
nullification, the probability for a single cycle of rotational perturbation to evade gravisensing in each cell would assume a broad distribution. As any putative biochemical gravisensing
reaction(s) would be stochastic, the probability for gravisensory evasion in each cell would decrease with both lower angular frequency and exposure to longer duration perturbations. We did
in fact observe such trends, as a result of the time lapse, single-cell measurement capabilities of the clinochip system. For our clinochips, we do not expect that centripetal forces have a
role with our observed angular frequency-dependence, as residual accelerations were calculated to be on the micro-scale regime. In addition, because the capillary number (indicative of
viscous forces) is orders of magnitude smaller than surface tension, fluid shear forces are deemed negligible. There is also no enhanced nutrient transport that accompanies clinorotation in
our system. Without these confounding factors, it seems reasonable to conclude that the cell morphology differences we observed were predominantly due to changes in the angular frequency of
clinorotation. We believe the rounding and retracted cell area of hMSCs in simulated microgravity inhibit migration in the presence of a chemical gradient. Interestingly, the kinetics of
cell area changes during retraction were different from those during spreading, and were also angular frequency independent, suggesting that distinct gravisensing mechanisms may regulate
different processes. Similarly, other cell processes, and even cell fate, could be affected.39 In the same way that microgravity may produce these cellular alterations, other mechanical
stimuli and substrate characteristics can also modulate cell function.40 Stem cell morphology has a strong correlation with cellular phenotype and differentiation potential.41,42 It has been
demonstrated in prior work that spread cells have a higher tendency to undergo osteogenesis and that cells with rounded morphologies are more susceptible to adipogenesis.42 Because of the
short-term nature of this current study, it is not possible to establish whether hMSCs in our system would behave according to these trends. However, our data would suggest that cells at 30
rpm clinorotation would be more amenable to osteogenic differentiation, whereas cells at 75 rpm would tend toward adipogenic or chondrogenic lineages. This may help explain the conflicting
reports on mesenchymal stem cells differentiation during clinorotation that we and others23 have observed. Future longer-term studies with the clinochip are required to explore hMSC
differentiation potential. Specifically, as we have shown that the population distribution of cell morphologies can be controlled by the angular frequency of rotation, we may be able to
derive cellular phenotypes desirable for stem cell renewal, repair, and tissue engineering. REFERENCES * Zwart SR, Morgan JL, Smith SM . Iron status and its relations with oxidative damage
and bone loss during long-duration space flight on the International Space Station. _Am J Clin Nutr_ 2013; 98: 217–223. Article CAS PubMed Google Scholar * LeBlanc A, Schneider V,
Shackelford L, West S, Oganov V, Bakulin A et al. Bone mineral and lean tissue loss after long duration space flight. _J Musculoskelet Neuronal Interact_ 2000; 1: 157–160. CAS PubMed
Google Scholar * Fitts RH, Trappe SW, Costill DL, Gallagher PM, Creer AC, Colloton PA et al. Prolonged space flight-induced alterations in the structure and function of human skeletal
muscle fibres. _J Physiol_ 2010; 588: 3567–3592. Article CAS PubMed PubMed Central Google Scholar * Whitson PA, Pietrzyk RA, Jones JA, Nelman-Gonzalez M, Hudson EK, Sams CF . Effect of
potassium citrate therapy on the risk of renal stone formation during spaceflight. _J Urol_ 2009; 182: 2490–2496. Article CAS PubMed Google Scholar * Morey ER, Baylink DJ . Inhibition of
bone formation during space flight. _Science_ 1978; 201: 1138–1141. Article CAS PubMed Google Scholar * Pittenger MF, Mackay AM, Beck SC, Jaiswal RK, Douglas R, Mosca JD et al.
Multilineage potential of adult human mesenchymal stem cells. _Science_ 1999; 284: 143–147. Article CAS PubMed Google Scholar * Bruder SP, Fink DJ, Caplan AI . Mesenchymal stem cells in
bone development, bone repair, and skeletal regeneration therapy. _J Cell Biochem_ 1994; 56: 283–294. Article CAS PubMed Google Scholar * Caplan AI, Dennis JE . Mesenchymal stem cells as
trophic mediators. _J Cell Biochem_ 2006; 98: 1076–1084. Article CAS PubMed Google Scholar * Luttges MW . Recognizing and optimizing flight opportunities with hardware and life sciences
limitations. _Trans Kans Acad Sci_ 1992; 95: 76–86. Article CAS PubMed Google Scholar * Huijser R, Aartman L, Willemsen H . Cells in space—sounding rocket facilities for cell biology
and biotechnology in microgravity. _Esa Sp Publ_ 1990; 307: 455–466. Google Scholar * Paul AL, Manak MS, Mayfield JD, Reyes MF, Gurley WB, Ferl RJ . Parabolic flight induces changes in gene
expression patterns in _Arabidopsis thaliana_. _Astrobiology_ 2011; 11: 743–758. Article CAS PubMed Google Scholar * van Loon JJWA . Some history and use of the random positioning
machine, RPM, in gravity related research. _Adv Space Res_ 2007; 39: 1161–1165. Article Google Scholar * Hemmersbach R, Strauch SM, Seibt D, Schuber M . Comparative studies on
gravisensitive protists on ground (2D and 3D clinostats) and in microgravity. _Microgravity Sci Tec_ 2006; 18: 257–259. Article Google Scholar * Klaus DM . Clinostats and bioreactors.
_Gravitat Space Biol Bull_ 2001; 14: 55–64. CAS Google Scholar * Lorenzi G, Perbal G . Root-growth and statocyte polarity in lentil seedling roots grown in microgravity or on a slowly
rotating clinostat. _Physiol Plant_ 1990; 78: 532–537. Article Google Scholar * Toy MF, Richard S, Kuhn J, Franco-Obregon A, Egli M, Depeursinge C . Enhanced robustness digital holographic
microscopy for demanding environment of space biology. _Biomed Opt Express_ 2012; 3: 313–326. Article PubMed PubMed Central Google Scholar * Yuge L, Kajiume T, Tahara H, Kawahara Y,
Umeda C, Yoshimoto R et al. Microgravity potentiates stem cell proliferation while sustaining the capability of differentiation. _Stem Cells Dev_ 2006; 15: 921–929. Article CAS PubMed
Google Scholar * Buravkova LB, Romanov YA . The role of cytoskeleton in cell changes under condition of simulated microgravity. _Acta Astronaut_ 2001; 48: 647–650. Article CAS PubMed
Google Scholar * Higashibata A, Imamizo-Sato M, Seki M, Yamazaki T, Ishioka N . Influence of simulated microgravity on the activation of the small GTPase Rho involved in cytoskeletal
formation--molecular cloning and sequencing of bovine leukemia-associated guanine nucleotide exchange factor. _BMC Biochem_ 2006; 7: 19. Article PubMed PubMed Central Google Scholar *
Dai ZQ, Wang R, Ling SK, Wan YM, Li YH . Simulated microgravity inhibits the proliferation and osteogenesis of rat bone marrow mesenchymal stem cells. _Cell Prolif_ 2007; 40: 671–684.
Article CAS PubMed PubMed Central Google Scholar * Yu B, Yu D, Cao L, Zhao X, Long T, Liu G et al. Simulated microgravity using a rotary cell culture system promotes chondrogenesis of
human adipose-derived mesenchymal stem cells via the p38 MAPK pathway. _Biochem Biophys Res Commun_ 2011; 414: 412–418. Article CAS PubMed Google Scholar * Sheyn D, Pelled G, Netanely D,
Domany E, Gazit D . The effect of simulated microgravity on human mesenchymal stem cells cultured in an osteogenic differentiation system: a bioinformatics study. _Tissue Eng Part A_ 2010;
16: 3403–3412. Article PubMed PubMed Central Google Scholar * Ulbrich C, Wehland M, Pietsch J, Aleshcheva G, Wise P, van Loon J et al. The impact of simulated and real microgravity on
bone cells and mesenchymal stem cells. _BioMed Res Int_ 2014; 2014: 928507. Article PubMed PubMed Central Google Scholar * Klaus DM, Todd P, Schatz A . Functional weightlessness during
clinorotation of cell suspensions. _Adv Space Res_ 1998; 21: 1315–1318. Article CAS PubMed Google Scholar * Yew AG, Atencia J, Hsieh AH . Lab-on-chip clinorotation system for live-cell
microscopy under simulated microgravity. _Cell Mol Bioeng_ 2014; 7: 165–170. Article Google Scholar * Ozaki Y, Nishimura M, Sekiya K, Suehiro F, Kanawa M, Nikawa H et al. Comprehensive
analysis of chemotactic factors for bone marrow mesenchymal stem cells. _Stem Cells Dev_ 2007; 16: 119–129. Article CAS PubMed Google Scholar * Quirici N, Soligo D, Bossolasco P, Servida
F, Lumini C, Deliliers GL . Isolation of bone marrow mesenchymal stem cells by anti-nerve growth factor receptor antibodies. _Exp Hematol_ 2002; 30: 783–791. Article CAS PubMed Google
Scholar * Wakabayashi K, Nagai A, Sheikh AM, Shiota Y, Narantuya D, Watanabe T et al. Transplantation of human mesenchymal stem cells promotes functional improvement and increased
expression of neurotrophic factors in a rat focal cerebral ischemia model. _J Neurosci Res_ 2010; 88: 1017–1025. CAS PubMed Google Scholar * Sokal RR, Rohlf FJ . Single classification
analysis of variance: finding the sample size required for a test. _Biometry_, 3rd (edn). W. H. Freeman and Co.: New York City, NY, USA, 1995; 260–265. Google Scholar * Son BR,
Marquez-Curtis LA, Kucia M, Wysoczynski M, Turner AR, Ratajczak J et al. Migration of bone marrow and cord blood mesenchymal stem cells _in vitro_ is regulated by stromal-derived
factor-1-CXCR4 and hepatocyte growth factor-c-met axes and involves matrix metalloproteinases. _Stem Cells_ 2006; 24: 1254–1264. Article CAS PubMed Google Scholar * Sarkar D, Nagaya T,
Koga K, Nomura Y, Gruener R, Seo H . Culture in vector-averaged gravity under clinostat rotation results in apoptosis of osteoblastic ROS 17/2.8 cells. _J Bone Miner Res_ 2000; 15: 489–498.
Article CAS PubMed Google Scholar * Baker PW, Meyer ML, Leff LG . Escherichia coli growth under modeled reduced gravity. _Microgravity Sci Technol_ 2004; 15: 39–44. Article PubMed
Google Scholar * Louis F, Deroanne C, Nusgens B, Vico L, Guignandon A . RhoGTPases as key players in mammalian cell adaptation to microgravity. _BioMed Res Int_ 2015; 2015: 747693. PubMed
PubMed Central Google Scholar * Sun Z, Cao X, Zhang Z, Hu Z, Zhang L, Wang H et al. Simulated microgravity inhibits L-type calcium channel currents partially by the up-regulation of
miR-103 in MC3T3-E1 osteoblasts. _Sci Rep_ 2015; 5: 8077. Article CAS PubMed PubMed Central Google Scholar * Kohn FPM . High throughput fluorescent screening of membrane potential and
intracellular calcium concentration under variable gravity conditions. _Microgravity Sci Tec_ 2013; 25: 113–120. Article CAS Google Scholar * Ng TL, Gown AM, Barry TS, Cheang MC, Chan AK,
Turbin DA et al. Nuclear beta-catenin in mesenchymal tumors. _Mod Pathol_ 2005; 18: 68–74. Article CAS PubMed Google Scholar * Li J, Zhang S, Chen J, Du T, Wang Y, Wang Z . Modeled
microgravity causes changes in the cytoskeleton and focal adhesions, and decreases in migration in malignant human MCF-7 cells. _Protoplasma_ 2009; 238: 23–33. Article PubMed Google
Scholar * Hammond TG, Hammond JM . Optimized suspension culture: the rotating-wall vessel. _Am J Physiol Renal Physiol_ 2001; 281: F12–F25. Article CAS PubMed Google Scholar * Matsuoka
F, Takeuchi I, Agata H, Kagami H, Shiono H, Kiyota Y et al. Morphology-based prediction of osteogenic differentiation potential of human mesenchymal stem cells. _PloS One_ 2013; 8: e55082.
Article CAS PubMed PubMed Central Google Scholar * Maul TM, Chew DW, Nieponice A, Vorp DA . Mechanical stimuli differentially control stem cell behavior: morphology, proliferation, and
differentiation. _Biomech Model Mechanobiol_ 2011; 10: 939–953. Article PubMed PubMed Central Google Scholar * Kilian KA, Bugarija B, Lahn BT, Mrksich M . Geometric cues for directing
the differentiation of mesenchymal stem cells. _Proc Natl Acad Sci USA_ 2010; 107: 4872–4877. Article CAS PubMed PubMed Central Google Scholar * McBeath R, Pirone DM, Nelson CM,
Bhadriraju K, Chen CS . Cell shape, cytoskeletal tension, and RhoA regulate stem cell lineage commitment. _Dev Cell_ 2004; 6: 483–495. Article CAS PubMed Google Scholar * Nishikawa M,
Ohgushi H, Tamai N, Osuga K, Uemura M, Yoshikawa H et al. The effect of simulated microgravity by three-dimensional clinostat on bone tissue engineering. _Cell Transplant_ 2005; 14: 829–835.
Article PubMed Google Scholar * Wu X, Li SH, Lou LM, Chen ZR . The effect of the microgravity rotating culture system on the chondrogenic differentiation of bone marrow mesenchymal stem
cells. _Mol Biotechnol_ 2013; 54: 331–336. Article CAS PubMed Google Scholar * Zhang X, Nan Y, Wang H, Chen J, Wang N, Xie J et al. Model microgravity enhances endothelium
differentiation of mesenchymal stem cells. _Naturwissenschaften_ 2013; 100: 125–133. Article CAS PubMed Google Scholar * Uddin SM, Qin YX . Enhancement of osteogenic differentiation and
proliferation in human mesenchymal stem cells by a modified low intensity ultrasound stimulation under simulated microgravity. _PloS One_ 2013; 8: e73914. Article CAS PubMed PubMed
Central Google Scholar * Zayzafoon M, Gathings WE, McDonald JM . Modeled microgravity inhibits osteogenic differentiation of human mesenchymal stem cells and increases adipogenesis.
_Endocrinology_ 2004; 145: 2421–2432. Article CAS PubMed Google Scholar * Saxena R, Pan G, McDonald JM . Osteoblast and osteoclast differentiation in modeled microgravity. _Ann N Y Acad
Sci_ 2007; 1116: 494–498. Article CAS PubMed Google Scholar * Meyers VE, Zayzafoon M, Gonda SR, Gathings WE, McDonald JM . Modeled microgravity disrupts collagen I/integrin signaling
during osteoblastic differentiation of human mesenchymal stem cells. _J Cell Biochem_ 2004; 93: 697–707. Article CAS PubMed Google Scholar * Chen J, Liu RR, Yang Y, Li J, Zhang XF, Li JC
et al. The simulated microgravity enhances the differentiation of mesenchymal stem cells into neurons. _Neurosci Lett_ 2011; 505: 171–175. Article CAS PubMed Google Scholar Download
references ACKNOWLEDGEMENTS FUNDING This work was supported by National Aeronautics and Space Administration Space Biology Program, Grant NNX13AM06G. AUTHOR INFORMATION AUTHORS AND
AFFILIATIONS * Fischell Department of Bioengineering, University of Maryland, College Park, MD, USA Carlos Luna & Adam H Hsieh * NASA Goddard Space Flight Center, Greenbelt, MD, USA
Alvin G Yew * Department of Orthopaedics, University of Maryland, Baltimore, MD, USA Adam H Hsieh Authors * Carlos Luna View author publications You can also search for this author inPubMed
Google Scholar * Alvin G Yew View author publications You can also search for this author inPubMed Google Scholar * Adam H Hsieh View author publications You can also search for this author
inPubMed Google Scholar CONTRIBUTIONS CL, AY, AH conceived the project and analyzed the data, CL performed the experiments, AY developed the lab-on-chip clinostat, and all authors
contributed to writing of the manuscript. CORRESPONDING AUTHOR Correspondence to Adam H Hsieh. ETHICS DECLARATIONS COMPETING INTERESTS The authors declare no conflict of interest. ADDITIONAL
INFORMATION Supplementary Information accompanies the paper on the _npj Microgravity_ (http://www.nature.com/npmgrav) SUPPLEMENTARY INFORMATION SUPPLEMENTARY FIGURE LEGENDS (DOC 26 KB)
SUPPLEMENTARY FIGURE 1 (JPG 1038 KB) SUPPLEMENTARY FIGURE 2 (JPG 320 KB) SUPPLEMENTARY FIGURE 3 (JPG 714 KB) SUPPLEMENTARY FIGURE 4 (JPG 546 KB) RIGHTS AND PERMISSIONS This work is licensed
under a Creative Commons Attribution-NonCommercial-NoDerivatives 4.0 International License. The images or other third party material in this article are included in the article’s Creative
Commons license, unless indicated otherwise in the credit line; if the material is not included under the Creative Commons license, users will need to obtain permission from the license
holder to reproduce the material. To view a copy of this license, visit http://creativecommons.org/licenses/by-nc-nd/4.0/ Reprints and permissions ABOUT THIS ARTICLE CITE THIS ARTICLE Luna,
C., Yew, A. & Hsieh, A. Effects of angular frequency during clinorotation on mesenchymal stem cell morphology and migration. _npj Microgravity_ 1, 15007 (2015).
https://doi.org/10.1038/npjmgrav.2015.7 Download citation * Received: 12 March 2015 * Revised: 15 May 2015 * Accepted: 12 June 2015 * Published: 30 July 2015 * DOI:
https://doi.org/10.1038/npjmgrav.2015.7 SHARE THIS ARTICLE Anyone you share the following link with will be able to read this content: Get shareable link Sorry, a shareable link is not
currently available for this article. Copy to clipboard Provided by the Springer Nature SharedIt content-sharing initiative