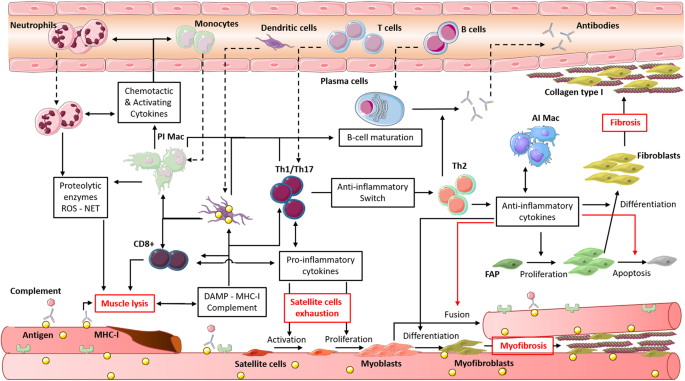
Stromal vascular fraction in the treatment of myositis
- Select a language for the TTS:
- UK English Female
- UK English Male
- US English Female
- US English Male
- Australian Female
- Australian Male
- Language selected: (auto detect) - EN
Play all audios:

ABSTRACT Muscle regeneration is a physiological process that converts satellite cells into mature myotubes under the influence of an inflammatory environment progressively replaced by an
anti-inflammatory environment, with precise crosstalk between immune and muscular cells. If the succession of these phases is disturbed, the immune system can sometimes become auto-reactive,
leading to chronic muscular inflammatory diseases, such as myositis. The triggers of these autoimmune myopathies remain mostly unknown, but the main mechanisms of pathogenesis are partially
understood. They involve chronic inflammation, which could be associated with an auto-reactive immune response, and gradually with a decrease in the regenerative capacities of the muscle,
leading to its degeneration, fibrosis and vascular architecture deterioration. Immunosuppressive treatments can block the first part of the process, but sometimes muscle remains weakened, or
even still deteriorates, due to the exhaustion of its capacities. For patients refractory to immunosuppressive therapies, mesenchymal stem cells have shown interesting effects but their use
is limited by their availability. Stromal vascular fraction, which can easily be extracted from adipose tissue, has shown good tolerance and possible therapeutic benefits in several
degenerative and autoimmune diseases. However, despite the increasing use of stromal vascular fraction, the therapeutically active components within this heterogeneous cellular product are
ill-defined and the mechanisms by which this therapy might be active remain insufficiently understood. We review herein the current knowledge on the mechanisms of action of stromal vascular
fraction and hypothesise on how it could potentially respond to some of the unmet treatment needs of refractory myositis. SIMILAR CONTENT BEING VIEWED BY OTHERS REGULATORY T CELLS IN
SKELETAL MUSCLE REPAIR AND REGENERATION: RECENT INSIGHTS Article Open access 05 August 2022 NON-MYOGENIC MESENCHYMAL CELLS CONTRIBUTE TO MUSCLE DEGENERATION IN FACIOSCAPULOHUMERAL MUSCULAR
DYSTROPHY PATIENTS Article Open access 16 September 2022 ADIPOSE TISSUE IS A SOURCE OF REGENERATIVE CELLS THAT AUGMENT THE REPAIR OF SKELETAL MUSCLE AFTER INJURY Article Open access 05
January 2023 FACTS * Muscle regeneration involves a sequence of tissue repair mechanisms regulated by both pro- and anti-inflammatory immune cells. * Myositis pathomechanisms are not fully
understood but may result from chronic exposure to immune cells and cytokines, leading to destruction and mis-repair of muscle, with fibrosis and vascular architecture disturbance. *
Therapeutic options for inflammatory myopathies are predominantly based on immunosuppressive treatments, which are sometimes insufficient to regulate the different features of these complex
pathologies. * Adipose tissue-derived stem cells and stromal vascular fraction have immunomodulatory, anti-fibrotic, proangiogenic and regenerative properties that could be exploited for the
treatment of refractory or relapsed myositis. * However, the mechanisms behind these effects are still insufficiently understood and more preclinical studies are required before their
clinical use. OPEN QUESTIONS * Does repeated acute muscular destruction trigger chronical autoimmune inflammation, or is auto-immunity responsible for muscle destruction in myositis? * In
myositis, how does chronicle inflammation affect adipose-derived cells capacities? * Will adipose stem cells replace muscle stem cells and directly participate in tissue regeneration, or
rather have a supporting role for immune and local stem cells? INTRODUCTION The mechanisms of muscle regeneration following injury are now well-known and involve both muscle and immune
cells, through a regulated process [1]. A disruption of this process may be the cause of chronic inflammation and a failure of muscle regeneration, which can lead to autoimmune diseases such
as myositis. Myositis, also called idiopathic inflammatory myopathies, represents a group of immune-mediated diseases, including Polymyositis (PM), Dermatomyositis (DM), Immune-Mediated
Necrotising Myositis (IMNM), Inclusion Body Myositis (IBM) and overlap myositis [2]. They are clinically characterised by muscle weakness, and histologically by the presence of varying
levels of myofibre necrosis and leucocyte infiltrates in muscles [3]. Predominantly muscular, some forms of myositis can also be associated with other manifestations, such as Interstitial
Lung Disease (ILD), skin ulcers or Raynaud’s phenomenon. Corticosteroids and immunosuppressive drugs are commonly used but may be ineffective in some patients or even aggravating due to
their possible side effects [4,5,6]. For these patients, cell therapies have sometimes shown long-term beneficial effects. Autologous hematopoietic stem cell transplants, which have been
used to replace auto-reactive immune systems, have improved patients’ conditions, and even allowed some of them to enter into remission. These therapies generally seem to be safe, but can be
complicated by severe or even life-threatening iatrogenic infections due to myeloablative conditioning regimens [7,8,9,10]. More recently, the discovery of the immunomodulatory effects of
Mesenchymal Stem Cells (MSC) from Bone Marrow (BM-MSC) or Umbilical Cord Blood (UC-MSC), in addition to their well-known regenerative effect, has led to their use for the treatment of
patients with refractory autoimmune diseases, including myositis [11]. If these types of stem cells seem safer, the invasiveness of the harvesting and the low rate of stem cells recovered
from these sources remain important limitations to their use. Adipose tissue is another source of MSC. They can be extracted safely and in large quantities from a lipoaspirate by enzymatic
digestion or mechanical isolation for Stromal Vascular Fraction (SVF), followed by replicative cultures of adherent cells for Adipose-Derived Stem Cells (ADSC) [12]. If ADSC are known to
possess immunomodulatory, proangiogenic, anti-fibrotic and regenerative capacities like MSC from other tissues [13], SVF share similar properties and is easier to prepare [14]. However, the
therapeutically active components within this heterogeneous cellular product are not well defined, and the mechanisms responsible for its activity remain insufficiently understood. In order
to evaluate the potential of SVF as a treatment for refractory myositis, we first summarise here the physiological mechanisms of muscle regeneration and the pathological mechanisms involved
in myositis. Next, we address the main features of these diseases through the known mechanisms of action of this cell therapy. Last, we discuss its clinical relevance by analysing results
from various clinical trials. PHYSIOLOGICAL MUSCLE REGENERATION Muscle regeneration after trauma is a process that involves both immune and muscular cells in order to restore normal muscle
function. At first, satellite cell activation and proliferation accompanied by inflammation, followed by a progressive decrease of inflammation under the influence of anti-inflammatory
cells, which stimulate muscle progenitor cell differentiation and tissue remodelling [15]. At the earliest stage of regeneration after injury, muscle damaged cells release Damage-Associated
Molecular Patterns (DAMP) [16], which lead through Toll Like Receptor (TLR) to the activation and infiltration of immune cells, mostly mast cells [17] and neutrophils [18]. These cells start
to clear the damaged myofibres and secrete pro-inflammatory cytokines (mostly IL-1, IL-6, IL-8 and TNFα). The pro-inflammatory signal spreads and after 24 h, macrophages can be observed at
the lesion site [19]. They are mostly involved in the elimination of damaged muscular cells by the production of Reactive Oxygen Species (ROS), through the increased expression of Inducible
Nitric Oxide Synthase (iNOS), and phagocytosis. Like neutrophils, they secrete a large amount of cytokines (mostly TNFα, IL-6, and IL-1β) which triggers a positive feedback loop between
neutrophil and macrophage recruitment and production of cytotoxic substances, but also T-cell recruitment [20]. Around three days after injury, both CD8+ and CD4+ T cells appear at the
lesion site and can be detected for up to ten days [21]. CD8+ T cells pursue the task of macrophages and neutrophils, by releasing many cytokines which amplify the recruitment of leucocytes
and by acting on extracellular matrix remodelling to speed up cellular debris elimination [22]. CD4+ T cells seem to be recruited a little later than CD8+ T cells [23]. They differentiate
preferentially into Th1 cells, which maintain macrophage recruitment and pro-inflammatory polarisation through the production of cytokines (IL-1β, TNFα and IFNγ). The large amount of
secreted cytokines modulates the environment of the injured site and triggers muscular regeneration [1]. Fu et al. demonstrated that the cytokines secreted by T cells promote satellite cell
proliferation in vitro and in vivo [24]. Indeed, TNFα is known to attract satellite cells to the damaged site and to enhance their proliferation through the activation of NF-κB signalling
and the p38 pathway [25, 26]. The importance of TNFα secretion in response to muscular damage has been demonstrated in vivo in TNFα or TNF-receptor knockout mice, which show severe muscular
regeneration defects [27, 28]. TNFα is associated with other cytokines, such as IL-6, secreted by both immune and muscle cells, and IL-1β, which maintains the proliferation but also
stimulates the differentiation of satellite cells into myoblasts [29, 30]. The importance of IL-1β has also been demonstrated in IL-1β knockout mice, which present a slowdown of satellite
cell differentiation, shown by a reduced expression of myoblast markers MyoD and Myogenin. When the clearing of damaged cells ends, the naive T cells recruited polarise into Th2 cells, which
release anti-inflammatory cytokines (IL-4 and IL-13) [31]. These cytokines stimulate myoblast fusion and Fibro–Adipogenic Progenitor (FAP) proliferation [32]. After activation and
differentiation, FAP are another source of myogenic factor like Wnt family members, IL-6 and Insulin-like Grow-Factor 1 (IGF1), which enhance satellite cell proliferation and myoblast
differentiation and fusion [33,34,35]. They also release IL-33, which participates in the activation of muscle Treg cells and the recruitment of circulatory Treg cells, which are genetically
and functionally distinct [36]. Indeed, in addition to regulating and reducing the inflammatory environment, muscle Treg cells play an important role in regeneration through amphiregulin
secretion [37, 38]. The change of inflammatory environment is mainly based on the change of macrophage populations from pro-inflammatory to anti-inflammatory, under the influence of Th2 and
Treg cell cytokines [39]. These macrophages produce anti-inflammatory cytokines (IL-4, IL-10 and IL-13), which reduce the local inflammation induced by the lesion and stimulate the
differentiation and fusion of myoblasts into myotubes, promoting the late stage of myogenesis [35, 40]. They also release TGFβ, which regulates myotube fusion and prevents the TNFα-induced
apoptosis of FAP, inducing their differentiation into matrix-producing cells [41]. These cells also produce growth factors such as IGF1, Hepatocyte Growth Factor (HGF), basic Fibroblast
Growth Factor (bFGF), and Vascular Endothelial Growth Factor (VEGF), which stimulate endothelial cells and contribute to bringing together endothelial and satellite cells [42]. The proximity
and crosstalk between muscular, mesenchymal and endothelial cells are essential for both myoangiogenesis and total muscle recovery [43]. DEFECTIVE MUSCLE REGENERATION DURING MYOSITIS The
myoregeneration process relies on the presence of functional satellite cells in the muscle and the environmental agents that control at different phases the proliferation, differentiation
and fusion of these cells into myotubes [44]. Among these stimuli, secretion by immune cells of pro- then anti-inflammatory cytokines plays an important role. In the case of myositis, a
chronic activation of innate and adaptive immune cells which can recognise auto-antigens is observed and these cells, normally transient, remain in the muscle (Fig. 1). Neutrophil
persistence in muscle infiltrates has been demonstrated in myositis muscle, as well as neutrophil participation in the destruction of muscle fibres through the release of proteolytic enzymes
and the formation of neutrophil extracellular traps [45, 46]. Further, this extracellular formation seems to be induced by the presence of myositis-associated antibodies. Reimann et al.
showed an increase in pro-inflammatory macrophage density in muscle, through iNOS expression correlated with a high expression of macrophage migration inhibitory factor, a cytokine with
anti-apoptotic, proliferative and chemotactic effects secreted by macrophages, T cells and muscle fibres [47]. Myositis patients present a strong Th1 response, with an increased expression
of IFNγ, IL-1β and TNFα [48, 49]. This Th1 response leads to the induction and maintenance of pro-inflammatory macrophages and thus amplifies muscle destruction [50]. A type 1 IFN signature
was observed in the blood of patients with DM or PM and correlated with disease activity [51]. A recent study showed that the activation of type 1 IFN pathway in muscle cells in vitro
induced myotube atrophy and impaired endothelial cell angiogenesis, features that were observed in DM [52]. Muscle destruction is also due to the abnormal expression of Major
Histocompatibility Complex type I (MHC-I) on the myocyte surface, induced by IFNγ and IL-1β [53]. This expression is also induced by IL-17, produced by Th17 cells, which potentiates the
effects of IL-1β. Some studies have shown that overexpression of MHC-I in muscle can induce myopathy through both immunological, with CD8+-mediated cytotoxicity, and nonimmunological
mechanisms [54, 55]. Thereby, both CD4+ and CD8+ T cells can be activated by muscle cells, which act directly as antigen presenting cells, but also indirectly through dendritic cells (DC)
detected in muscular infiltrate during myositis [56], or TLR stimulation by DAMP [16, 57]. The activation and differentiation of T cells by these cells lead to the formation of a permissive
environment for B-cell maturation, evidenced by the presence of CD19+ or CD20+ B cells and CD138+ plasma cells and by the expression of B-cell activating factor [58]. Myositis specific
antibodies were identified in more than half of patients [2], and a recent study associated Th1 and Th17 cytokine expression with B-cell aggregation and maturation, through the formation of
ectopic lymphoid structures in myositis muscle [59]. The pathogenicity of these antibodies has not always been demonstrated, however they are often correlated with the severity of the
myositis and the underlying diseases, like ILD, Raynaud syndrome or cancer [60]. Furthermore for some of them, the mechanism of action has been identified, notably in IMNM, wherein
anti-3-Hydroxy-3-methylglutaryl-CoA reductase (HMGCR) and anti-signal recognition protein (SRP) induce myofibre atrophy by increasing IL-6, TNFα and ROS secretion. They also reduce myotube
formation by decreasing IL-4 and IL-13 production [61]. This pathogenic effect seems also to involve complement system [62]. Anti-inflammatory cells are also present in myositis muscle and
some of them take part in the pathomechanism. If the Th2 cytokine IL-4 overexpressed in myositis seems inversely correlated with the severity of muscular destruction, as evidenced by
muscular enzyme levels in the sera [63], anti-inflammatory macrophage cytokines (TGFβ and IL-10) are also highly expressed in myositis and seem to be involved in the pathogenesis [47, 64].
Prolonged exposure to TGFβ and IL-10 is correlated with the duration of myositis via their association with other cytokines, especially in IBM [50, 65]. Prolonged TGFβ1 exposure, for
example, is strongly involved in the dysregulation of muscle regeneration in different myopathies, by inhibiting satellite cell activation and differentiation and myocyte fusion, but also by
increasing myofibroblast accumulation and fibrosis [66,67,68]. Muscle fibrosis is also generated by the accumulation of FAP, which escape TNF-induced apoptosis and differentiate into
collagen type-1 producing cells (fibroblasts) under TGFβ1 high expression by anti-inflammatory macrophages [41, 69]. This escape from apoptosis could also be due to the expression of immune
checkpoint factors (PD-L1, PD-L2 and CD47) by FAP, as shown in a murine model of myositis [70]. Fibrosis can lead, like in IBM, to tissue and capillary architecture disruption and to an
increased distance between muscle fibres and capillaries, responsible for hypoxia and oxidative stress [71]. Treg cell functions are also altered in myositis, which leads to the
dysregulation of immune response and to the impairment of muscle regeneration. Indeed, Treg dysfunctions have been evidenced in both DM and PM and in several myositis models, which present a
more severe disease when Treg cells are depleted. Conversely, the injection of functional Treg cells at the time of immunisation blocked disease progression [72]. On the other hand, Treg
cells seem to be fully functional in IBM despite a decreased frequency observed in circulation and muscle [49]. If the pathogenic triggers of myositis have not yet been identified, partially
due to a lack of spontaneous animal models, the hypothesis of an immune system defect which is solely responsible or even at the origin of the disease is controversial, especially in IBM
[73, 74]. Muscle cells participate in the pathogenesis and aggravation of the disease, through the activation of several deleterious and not fully understood mechanisms, involving
endoplasmic reticulum and/or mitochondrial defects. If MHC-I expression on muscle cell surface is often linked to leucocyte infiltration, it has been shown that it can be induced by
endoplasmic reticulum stress, and that its presence can be detected before infiltrate and can affect muscle fibre contractibility [75]. Beyond muscle destruction, which stays chronically
blocked in pro- and/or anti-inflammatory state without resolution, the impairment of satellite cell capacities to activate and proliferate is also involved in the disease. Myoblasts from IBM
patients also present reduced proliferation rate and clonogenicity capacity in vitro when compared to myoblasts from healthy donors, probably due to replicative exhaustion and senescence
[76]. This observation was confirmed in vivo, with a lower expression of MyoD [71]. Moreover, the persistence of myogenin expression observed in these patients might reflect an impairment of
both myoblast differentiation and muscle fibre maturation. Myositis represents a heterogeneous group of diseases which involve immune and non-immune mechanisms. In these diseases, the
immune system acts on both muscle degeneration and regeneration, with muscle progenitor cell stimulation by cytokines. While suppressing myositis may appear as an effective solution to stop
or to slow down the disease, this can be insufficient or even aggravating sometimes, due to non-immune mechanisms or regenerative defects. CURRENT THERAPEUTICS FOR MYOSITIS PATIENTS Most of
the treatments used in myositis target the inflammatory actors. Glucocorticoids are the most current therapeutic approach, alone as a first-line treatment or associated with other
immunosuppressive molecules in refractory patients [77]. These associations are more efficient to improve muscle function, and act on myositis-associated diseases like ILD. Moreover, they
allow to reduce the corticosteroid effective dose, leading to fewer side effects, especially on muscle [78]. Nevertheless, the use of immunosuppressive molecules is also limited by their own
adverse effects, including toxicities on various organs (liver, lung, kidney, heart), risk of infection and metabolic disorders (diabetes, dyslipidemia) [79]. These various side effects are
even more problematic in elderly subjects who suffer from numerous co-morbidities. Biological agents have also been tested in myositis: at first, Intravenous (IV) immunoglobulins,
considered to present immunomodulatory properties, and then monoclonal antibodies, such as Rituximab (anti-CD20), Tocilizumab (anti-IL-6), Infliximab (anti-TNFα), Alemtuzumab (anti-CD52),
Basiliximab (anti-IL-2R) or Sifalimumab (anti-IFNα), or even receptor antagonist Anakinra (anti-IL-1R), JAK inhibitor Tofacitinib and fusion proteins Abatacept or Etanercept, which block
CTLA-4 or TNFα pathways, respectively [6, 80, 81]. If these treatments are efficient in most of first- and second-lines refractory to DM and PM, some patients stay refractory or relapse to
all of them. Complement inhibition has also been tested in IMNM, with a preventive effect in animals but no significant improvement in management in humans [82, 83]. Further, these
treatments seem to bring no benefit and even to exacerbate the disease in IBM patients, who remain in a therapeutic deadlock [4]. In this myositis subtype, new molecules are under
evaluation, like Arimoclomol, a Heat Shock Factor 1 activation amplificator, which increases chaperone protein activity and thus misfolding protein repair pathway, or follistatin, a
myostatin antagonist, with an adeno-associated virus coding for it injected Intramuscularly (IM) [84]. New monoclonal antibodies targeting myostatin or activin A, which both negatively
regulate myogenesis, have been developed but clinical trials in IBM have been recently withdrawn or cancelled for these treatments [85]. Cell therapies are occasionally used to treat
patients with refractory PM or DM, but very few cases have been reported. As stated above, hematopoietic stem cell autologous transplantation, used to replace auto-reactive immune system
after its depletion, has shown benefits but with a high risk of side effects [7,8,9,10]. MSC transplantations, for which no myeloablative treatment is required, have also been tested (Table
1). The first clinical study using MSC was published in 2011 by Wang et al. and reported the case of 10 patients with refractory PM or DM, according to Bohan and Peter criteria [86]. After
treatment by IV allogeneic BM- or UC-MSC, they observed an improvement in muscle strength and clinical score and a reduction of inflammation and muscle degeneration [87]. Transplanted cells
were also effective on associated symptoms, notably ILD and skin ulcer, and allowed the reduction of immunosuppressive baseline treatments for all patients. However, three patients relapsed
and two patients died from aggravation of a respiratory tract infection. This cohort was followed-up and has grown overtime to thirty patients and safety data were published in 2018. They
showed a low frequency of hyperacute adverse events, but reported the death of 11 patients from their underlying diseases, mostly ILD [11]. Lai et al. published the only controlled clinical
trial, comparing conventional therapy (6 months of corticosteroids and immunosuppressors) with (_n_ = 37 patients) or without (_n_ = 44 patients) allogeneic UC-MSC injections in patients
with PM or DM [88]. After a follow-up of 6–12 months, the authors demonstrated an improved efficacy of conventional therapy by MSC infusion on both clinical features and muscle strength (MMT
score), confirmed by a decrease in plasma creatine kinase level. They also showed an effect on associated ILD, with a reduction of interstitial pulmonary lesions on high resolution CT scan.
Clinical and biological controls at 1, 3 and 6 months after transplantation did not reveal any complications. Only one study reported the case of a patient with refractory and disabling PM
treated by four ADSC infusions, leading to an improvement in muscle strength (MMT score) and mobility 3 months after treatment, but no significant change in blood laboratory values [89]. The
patient continued corticosteroid treatment at a lower dose. Pharmaceutical and biological treatments have evolved and now allow to treat most of patients with myositis. After multiple lines
and associations of treatment, some patients with PM or DM remain refractory and more often those with IBM. In the latter case, new treatments under evaluation target more specifically
muscle degeneration, through metabolic or regulatory pathways, but the expected effect is not always obtained. Conversely, MSC therapies have shown interesting results in myositis, but their
harvest and preparation are not simple and clinical studies remain rare. SVF, which has similar effects, could be an alternative, provided its clinical efficacy is proved through robust
clinical trials. Two of them are about to start to study the safety of SVF in IBM (NCT04975841, NCT05032131). STROMAL VASCULAR FRACTION IN CLINICAL PRACTICE SVF isolation consists in
adipocyte elimination through enzymatic or mechanical procedures [90, 91]. Due to the variety of isolation methods, the International Federation for Adipose Therapeutics and Science (IFATS)
and the International Society for Cellular Therapy (ISCT) have released a joint statement that defines phenotypic and functional criteria for the identification of adipose-derived cells, and
proposes a general composition for SVF [12]: MSC (15–30%), Endothelial Progenitor Cells (EPC) (10–20%), Pericytes (3–5%) and leucocytes (25–45%) (Fig. 2A). According to the SVF isolation
method used, two types of SVF can be defined: cellular SVF, which can be obtained by enzymatic procedure, and tissular SVF, which is mechanically isolated and preserves cell-cell contact and
extracellular matrix [92, 93]. Recently, another intermediate type has been described: adipose-derived microvascular fraction which consists in lipoaspirate cells enzymatically digested for
a shorter time than cellular SVF, and which contains intact arteriolar, capillary and venous vessel segments [94]. Today, SVF is widely used and frequently compared to ADSC in both
regenerative medicine and immune/inflammatory disorders and presents significant advantages. Indeed, SVF extraction is simpler and quicker than ADSC expansion and does not present the risks
associated with long-term cell culture (bacterial or fungal contaminations, muta- or tumorigenesis). Combined with a permissive regulatory framework in some countries, the use of SVF has
regrettably been diverted by private clinics with little interest in studying the efficacy and mechanisms of action of this therapy, which has slowed its development. This was particularly
observed in the United States, which had an unusually low number of publications on the topic [95] whereas a very large number of clinics offer these unproven therapies [96, 97]. However,
this situation is about to change, with the end of the Food and Drug Administration discretion policy period which should enforce manufacturers, clinics, and health care practitioners
compliance with the new guidance on the regulatory framework for regenerative medicine therapies and ensure a greater safety for the use of these therapies [98]. The use of SVF has been
reported in many indications and it may represent a promising adjunctive therapy for patients with diseases for which current and conventional therapies are inadequate. The variety of these
indications was recently reviewed by Andia et al. who analysed 71 published clinical studies evaluating SVF [99]. However, more than 65% of them were case series with a low level of
evidence, and only 16% of them were randomised clinical trials. To confirm this analysis, we searched for clinical trials on clinicaltrial.gov database in May 2023 (search strategy with the
key words: Stromal vascular fraction OR SVF OR ADSVF OR ADRC OR ADSC), which allowed us to identify 169 clinical trials evaluating SVF injection (Fig. 2B). The most common indications,
according to these studies, were orthopaedic disorders (50.7% and 28.9% for published studies and clinical trials, respectively), then chronic wounds (14% and 24.1%) and cardiovascular and
pulmonary diseases (12.6% and 13.9%) (Fig. 2B). Other frequent indications were urogenital (5.6% and 9.6%), neurological (5.6% and 4.8%), and autoimmune diseases (5.6% and 11%), which often
overlapped with chronic wound treatment. These data are consistent with those from other studies [14, 99] and demonstrate the increasing interest of SVF for the treatment of inflammatory,
degenerative or non-regenerative, autoimmune or cardiovascular diseases. Local sites of injection were more frequent than intravascular, with a predominance of sub-cutaneous,
intra-articular, intra-fistula and intramuscular injection (Fig. 2D). Nevertheless, SVF is still a new therapy under development and clinical trials were mostly pilot studies in phase I (73%
versus 32.9% for all clinical trials), and phase II and III appeared only recently (23% versus 42.4% and 4% versus 24.7%, respectively) (Fig. 2E). Furthermore, several SVF clinical trials
were not completed (39% versus 15%), closed prematurely or withdrawn (23.3% versus 4.3%) (Fig. 2F). None of these closures were due to a safety issue. Some recent studies reviewed adverse
events in patients receiving “unproven or unapproved” stem cell therapies reported in scientific publications, clinical case reports and also mass media publications, and showed an increased
number of severe complications and hospitalisations compared to conventional therapies [100, 101]. Conversely, very few treatment‐related adverse events were noted during clinical trials,
demonstrating the safety of this procedure. Recently, clinical study results published for both SVF and ADSC were reviewed and showed that the most frequent and severe adverse events were
immunological and thromboembolic. They concerned predominantly ADSC, which were more frequently used in an allogeneic context and via IV injection than SVF [95]. Indeed, the in vitro
expansion of ADSC led to an increase in cell size, which significantly increased the risk of vascular obstruction and cerebral or myocardial stroke [102]. The injection of SVF directly at
the lesion site or within organs seems to be a safer way to use this therapy. Intramuscular injections of SVF were clinically used as a local route for the treatment of muscle sequelae [103,
104], allowing an improvement in muscle strength, or for the treatment of limb ischaemia [105, 106], highlighting its proangiogenic effects. In these studies, the safety of this route was
confirmed. Furthermore, IM injection led to the release of paracrine effectors in blood circulation and could be an alternative to other injection sites (intrathecal, intra-articular) [107].
Another advantage of this route was the increased dwell time of the injected cells, increasing from days to months the persistence in the body of these cells [108]. Even if they remained in
the muscle, they still responded to distant inflammatory signals and acted on distant sites [109]. IM injection of SVF could lead to prolonged clinical efficacy compared to other routes, in
both injected and non-injected muscles. POTENTIAL MECHANISMS OF ACTION OF STROMAL VASCULAR FRACTION IN MYOSITIS As seen above, intramuscular injection of SVF is known to be safe and has
promising clinical effects in both autoimmune and muscular diseases. Its potential interest in myositis treatment is based on four properties, but mechanisms of action are not fully known
(Fig. 3). First, the immunomodulatory effect of SVF is supported mainly by three cell populations: AD-MSC, macrophages and Treg cells. AD-MSC immunomodulatory capacities are similar or
higher to those of BM-MSC according to different studies [110, 111] and have already been tested in vitro [112] and in many in vivo models [113]. Even if the mechanisms involved are not
fully understood for these cells, several studies support their effects on T-cell activation, proliferation and differentiation from Th1 cells into Th2 cells, through soluble factors like
PGE2 and IDO [114,115,116] and direct interactions via CD54/CD2 and CD58/CD11a, which increase IL-10 production [117]. They also induce Treg proliferation, via TGFβ and IL-33 secretion [118,
119], and few adipose tissue Tregs are present in SVF. MSC also act on macrophage anti-inflammatory switch, in part by the secretion of PGE2 and IDO [120] and the interaction of CD90 and
CD11b trapping monocytes and macrophages into an anti-inflammatory niche [121]. Recent studies have shown that the interactions between MSC and pro-inflammatory cells enhance the
immunosuppressive capacities of MSC. Indeed, these authors observed that IFNγ and TNFα secreted by Th1 lymphocytes or CD54 expressed by pro-inflammatory macrophages increased IDO activities
[122, 123]. SVF also contains macrophages which exhibit anti-inflammatory activities through the secretion of high levels of IL-10 and IL-1 decoy receptors [124] that attenuate TNFα
inflammatory signals via activation of STAT3 [125, 126], and modulation of inflammatory gene transcription rates [127]. The modification of the balance between Arg-1 and iNOS activities,
which both use L-arginine as a substrate, leads to decreased ROS production, and thus to reduced oxidative stress and destruction of myofibres. ADSC and SVF can also act on fibrosis via
their immunomodulatory effects. Indeed, by reprogramming immune cells into anti-inflammatory cells, they increase the expression of IL-10, which presents several anti-fibrotic properties:
inhibition of neutrophil and macrophage invasion and ROS release [128], down-regulation of TGFβ1 expression [129], up-regulation of MMP and down-regulation of collagen expression [130].
Preclinical and clinical studies suggest that SVF anti-fibrotic effects are strongly related to the secretion of HGF by MSC during inflammatory responses, as evidenced by clinical and
histological parameters [131, 132]. Indeed, through the paracrine effect of this factor, SVF and ADSC reduce the expression of TGFβ1 and thus the differentiation of collagen type I/III
producing cells (fibroblasts) and alpha-Smooth Muscle Actin producing cells (myofibroblasts). ADSC also induce a significant increase in TGFβ3, which reduces the expression of these genes,
and stimulates MMP-1, -2 and -3 expressions, which increase fibrotic molecule degradation. The change in the TGFβ1/TGFβ3 and MMP-2/TIMP-2 ratio tips the scales in favour of an anti-fibrotic
effect [133, 134]. MMP expression is also stimulated by proangiogenic factors, like bFGF or VEGF, to degrade extracellular matrix and prepare neo-angiogenesis [135]. Indeed, SVF is known to
express high levels of IGF1, IL-8, Platelet-Derived Growth Factor-beta (PDGFβ), bFGF and VEGF, and to have robust angiogenic and vasculogenic activities demonstrated both in vitro and in
vivo in a hind limb ischaemia model [136]. These growth factors help to maintain a vascular-like micro-environment that supports MSC differentiation into endothelial cells, and thus
participate in angiogenesis and vascular repair during muscular regeneration [137]. Traktuev et al. demonstrated that VEGF helps the migration of MSC and promotes the secretion of PDGFβ by
EPC, which enable MSC to proliferate [138, 139]. PDGFβ is well-known for its action during vascular development [140] but also plays a role in the proangiogenic properties of SVF, by
inducing the secretion of proangiogenic extracellular vesicles by both MSC and EPC [141, 142]. These extracellular vesicles contain proangiogenic molecules such as c-KIT and Stem cell
factor, which participate in the recruitment of EPC and their differentiation into endothelial cells [143]. PDGFβ secretion by EPC also induces pericyte recruitment [144] which is known to
play an essential role in angiogenesis regulation [145]. SVF, through its immunomodulatory properties, acts on both chronic inflammation and muscle repair via cytokine release (mainly IL-4
and IL-13). Moreover, growth factors secreted by stromal cells may have a positive effect on muscle regeneration, and some of them are currently under evaluation in the management of muscle
disorders, such as sarcopenia [146]. But one advantage to using cell therapy, rather than hormones or cytokines, could be its ability to differentiate in situ depending on its cellular
environment. This could strengthen and help satellite cells to replace defective cells. The conversion of ADSC or SVF to a myogenic phenotype has been obtained in vitro by addition of
inductive media, containing horse serum and hydrocortisone. This leads to the expression of the myogenic transcription factors Myod1 and myogenin and then the fusion and formation of
multinucleated cells expressing the myosin heavy chain [147,148,149]. Based on histological evidence, ADSC fuse to form multinucleated myotubes in vitro. In their study, Di Rocco et al.
showed that ADSC and SVF cells were able to differentiate into skeletal muscle cells when cultured in the presence of differentiating primary myoblasts [150]. Furthermore, the conversion of
SVF to a myogenic phenotype is enhanced by myogenic environment in the absence of cell-cell contacts (transwell culture) and even in absence of muscle cells but to a lesser extent. This
myogenic conversion has also been demonstrated in vivo by several studies. In a lagomorphic model of muscular injury induced by cardiotoxin, the intramuscular injection of short-term
cultured (3 days) SVF cells induced an increase in muscle mass and functional capacities [151]. The myogenic differentiation of SVF and fusion with muscular cells have been demonstrated
using SVF genetically modified to express β-galactosidase or GFP, showing evidence of the contribution of SVF cells to muscular regeneration in vivo with 20% of GFP-positive fibres in the
total area of sections from treated hind limbs [150, 151]. This contribution could be enhanced by pretreating SVF with anti-inflammatory cytokine IL-4 or SDF1 before use to increase the
myogenic capacity of ADSC in vitro and in vivo [152]. To finish, it has also been reported that injection of human ADSC into immunocompetent mdx mice resulted in a substantial expression of
human dystrophin in both injected and adjacent muscle, revealing the spread of cells to other muscles [153]. CONCLUSION MSC-based therapies have shown interesting effects in the treatment of
refractory myositis, but their clinical use remains limited, especially for those extracted from adipose tissue. However, SVF, easily harvested from this tissue, could be beneficial for
patients thanks to its properties combining an immunomodulatory effect and a response to the main muscular complications of myositis. While published cases report only IV infusion of MSC in
myositis treatment, IM injection of SVF seems to be an interesting alternative, providing both local and systemic effects. Taken together, the evidence reviewed here seems to predict a
potential benefit of SVF in myositis treatment. However, these findings also highlight the need for preclinical studies and clinical trials to better understand the mechanisms of this
therapy and to optimise the practical modalities to ensure its safety and efficacy. REFERENCES * Yang W, Hu P. Skeletal muscle regeneration is modulated by inflammation. J Orthop Transl.
2018;13:25–32. Google Scholar * Allenbach Y, Benveniste O, Goebel H-H, Stenzel W. Integrated classification of inflammatory myopathies. Neuropathol Appl Neurobiol. 2017;43:62–81. CAS
PubMed Google Scholar * Meyer A, Lannes B, Goetz J, Echaniz-Laguna A, Lipsker D, Arnaud L, et al. Inflammatory myopathies: a new landscape. Jt Bone Spine. 2018;85:23–33. CAS Google
Scholar * Benveniste O, Guiguet M, Freebody J, Dubourg O, Squier W, Maisonobe T, et al. Long-term observational study of sporadic inclusion body myositis. Brain J Neurol. 2011;134:3176–84.
Google Scholar * Jabari D, Vedanarayanan VV, Barohn RJ, Dimachkie MM. Update on inclusion body myositis. Curr Rheumatol Rep. 2018;20:52. PubMed Google Scholar * Oddis CV, Aggarwal R.
Treatment in myositis. Nat Rev Rheumatol. 2018;14:279–89. CAS PubMed Google Scholar * Baron F, Ribbens C, Kaye O, Fillet G, Malaise M, Beguin Y. Effective treatment of Jo-1-associated
polymyositis with T-cell-depleted autologous peripheral blood stem cell transplantation. Br J Haematol. 2000;110:339–42. CAS PubMed Google Scholar * Zhu J, Su G, Lai J, Dong B, Kang M, Li
S, et al. Long-term follow-up of autologous hematopoietic stem cell transplantation for refractory juvenile dermatomyositis: a case-series study. Pediatr Rheumatol. 2018;16:72. Google
Scholar * Henes JC, Heinzelmann F, Wacker A, Seelig HP, Klein R, Bornemann A, et al. Antisignal recognition particle-positive polymyositis successfully treated with myeloablative autologous
stem cell transplantation. Ann Rheum Dis. 2009;68:447–8. CAS PubMed Google Scholar * Salvador FB, Isenberg DA. Use of autologous stem cell transplantation in adult patients with
idiopathic inflammatory myopathies: a case-report and review of the literature. Clin Exp Rheumatol. 2012;30:979. PubMed Google Scholar * Liang J, Zhang H, Kong W, Deng W, Wang D, Feng X,
et al. Safety analysis in patients with autoimmune disease receiving allogeneic mesenchymal stem cells infusion: a long-term retrospective study. Stem Cell Res Ther. 2018;9:312. PubMed
PubMed Central Google Scholar * Bourin P, Bunnell BA, Casteilla L, Dominici M, Katz AJ, March KL, et al. Stromal cells from the adipose tissue-derived stromal vascular fraction and culture
expanded adipose tissue-derived stromal/stem cells: a joint statement of the International Federation for Adipose Therapeutics and Science (IFATS) and the International Society for Cellular
Therapy (ISCT). Cytotherapy. 2013;15:641–8. PubMed PubMed Central Google Scholar * Zuk PA, Zhu M, Mizuno H, Huang J, Futrell JW, Katz AJ, et al. Multilineage cells from human adipose
tissue: implications for cell-based therapies. Tissue Eng. 2001;7:211–28. CAS PubMed Google Scholar * Bora P, Majumdar AS. Adipose tissue-derived stromal vascular fraction in regenerative
medicine: a brief review on biology and translation. Stem Cell Res Ther. 2017;8:145. PubMed PubMed Central Google Scholar * Howard EE, Pasiakos SM, Blesso CN, Fussell MA, Rodriguez NR.
Divergent roles of inflammation in skeletal muscle recovery from injury. Front Physiol. 2020;11:87. PubMed PubMed Central Google Scholar * Bianchi ME, Manfredi AA. High-mobility group box
1 (HMGB1) protein at the crossroads between innate and adaptive immunity. Immunol Rev. 2007;220:35–46. CAS PubMed Google Scholar * Gorospe JR, Nishikawa BK, Hoffman EP. Recruitment of
mast cells to muscle after mild damage. J Neurol Sci. 1996;135:10–17. CAS PubMed Google Scholar * Fielding RA, Manfredi TJ, Ding W, Fiatarone MA, Evans WJ, Cannon JG. Acute phase response
in exercise. III. Neutrophil and IL-1 beta accumulation in skeletal muscle. Am J Physiol. 1993;265:R166–72. CAS PubMed Google Scholar * Butterfield TA, Best TM, Merrick MA. The dual
roles of neutrophils and macrophages in inflammation: a critical balance between tissue damage and repair. J Athl Train. 2006;41:457–65. PubMed PubMed Central Google Scholar * Arango
Duque G, Descoteaux A. Macrophage cytokines: involvement in immunity and infectious diseases. Front Immunol. 2014;5:491. PubMed PubMed Central Google Scholar * Cheng M, Nguyen M-H,
Fantuzzi G, Koh TJ. Endogenous interferon-gamma is required for efficient skeletal muscle regeneration. Am J Physiol Cell Physiol. 2008;294:C1183–91. CAS PubMed Google Scholar * Zhang J,
Xiao Z, Qu C, Cui W, Wang X, Du J. CD8 T cells are involved in skeletal muscle regeneration through facilitating MCP-1 secretion and Gr1(high) macrophage infiltration. J Immunol.
2014;193:5149–60. CAS PubMed Google Scholar * Madaro L, Bouché M. From innate to adaptive immune response in muscular dystrophies and skeletal muscle regeneration: the role of
lymphocytes. BioMed Res Int. 2014;2014:438675. PubMed PubMed Central Google Scholar * Fu X, Xiao J, Wei Y, Li S, Liu Y, Yin J, et al. Combination of inflammation-related cytokines
promotes long-term muscle stem cell expansion. Cell Res. 2015;25:655–73. CAS PubMed PubMed Central Google Scholar * Peterson JM, Bakkar N, Guttridge DC. NF-κB signaling in skeletal
muscle health and disease. Curr Top Dev Biol. 2011;96:85–119. CAS PubMed Google Scholar * Chen S-E, Jin B, Li Y-P. TNF-alpha regulates myogenesis and muscle regeneration by activating p38
MAPK. Am J Physiol Cell Physiol. 2007;292:C1660–71. CAS PubMed Google Scholar * Chen S-E, Gerken E, Zhang Y, Zhan M, Mohan RK, Li AS, et al. Role of TNF-{alpha} signaling in regeneration
of cardiotoxin-injured muscle. Am J Physiol Cell Physiol. 2005;289:C1179–87. CAS PubMed Google Scholar * Warren GL, Hulderman T, Jensen N, McKinstry M, Mishra M, Luster MI, et al.
Physiological role of tumor necrosis factor alpha in traumatic muscle injury. FASEB J. 2002;16:1630–2. CAS PubMed Google Scholar * Muñoz-Cánoves P, Scheele C, Pedersen BK, Serrano AL.
Interleukin-6 myokine signaling in skeletal muscle: a double-edged sword? FEBS J. 2013;280:4131–48. PubMed PubMed Central Google Scholar * Chaweewannakorn C, Tsuchiya M, Koide M,
Hatakeyama H, Tanaka Y, Yoshida S, et al. Roles of IL-1α/β in regeneration of cardiotoxin-injured muscle and satellite cell function. Am J Physiol Regul Integr Comp Physiol.
2018;315:R90–103. CAS PubMed Google Scholar * Gordon S. Alternative activation of macrophages. Nat Rev Immunol. 2003;3:23–35. CAS PubMed Google Scholar * Heredia JE, Mukundan L, Chen
FM, Mueller AA, Deo RC, Locksley RM, et al. Type 2 innate signals stimulate fibro/adipogenic progenitors to facilitate muscle regeneration. Cell. 2013;153:376–88. CAS PubMed PubMed Central
Google Scholar * Sciorati C, Rigamonti E, Manfredi AA, Rovere-Querini P. Cell death, clearance and immunity in the skeletal muscle. Cell Death Differ. 2016;23:927–37. CAS PubMed PubMed
Central Google Scholar * Otto A, Schmidt C, Luke G, Allen S, Valasek P, Muntoni F, et al. Canonical Wnt signalling induces satellite-cell proliferation during adult skeletal muscle
regeneration. J Cell Sci. 2008;121:2939–50. CAS PubMed Google Scholar * Serrano AL, Baeza-Raja B, Perdiguero E, Jardí M, Muñoz-Cánoves P. Interleukin-6 is an essential regulator of
satellite cell-mediated skeletal muscle hypertrophy. Cell Metab. 2008;7:33–44. CAS PubMed Google Scholar * Burzyn D, Kuswanto W, Kolodin D, Shadrach JL, Cerletti M, Jang Y, et al. A
special population of regulatory T cells potentiates muscle repair. Cell. 2013;155:1282–95. CAS PubMed PubMed Central Google Scholar * Kuswanto W, Burzyn D, Panduro M, Wang KK, Jang YC,
Wagers AJ, et al. Poor repair of skeletal muscle in aging mice reflects a defect in local, interleukin-33-dependent, accumulation of regulatory T cells. Immunity. 2016;44:355–67. CAS PubMed
PubMed Central Google Scholar * Wu J, Ren B, Wang D, Lin H. Regulatory T cells in skeletal muscle repair and regeneration: recent insights. Cell Death Dis. 2022;13:680. PubMed PubMed
Central Google Scholar * Stout RD, Suttles J. Functional plasticity of macrophages: reversible adaptation to changing microenvironments. J Leukoc Biol. 2004;76:509–13. CAS PubMed Google
Scholar * Ruffell D, Mourkioti F, Gambardella A, Kirstetter P, Lopez RG, Rosenthal N, et al. A CREB-C/EBPbeta cascade induces M2 macrophage-specific gene expression and promotes muscle
injury repair. Proc Natl Acad Sci USA. 2009;106:17475–80. CAS PubMed PubMed Central Google Scholar * Lemos DR, Babaeijandaghi F, Low M, Chang C-K, Lee ST, Fiore D, et al. Nilotinib
reduces muscle fibrosis in chronic muscle injury by promoting TNF-mediated apoptosis of fibro/adipogenic progenitors. Nat Med. 2015;21:786–94. CAS PubMed Google Scholar * Pillon NJ, Bilan
PJ, Fink LN, Klip A. Cross-talk between skeletal muscle and immune cells: muscle-derived mediators and metabolic implications. Am J Physiol Endocrinol Metab. 2013;304:E453–65. CAS PubMed
Google Scholar * Christov C, Chrétien F, Abou-Khalil R, Bassez G, Vallet G, Authier F-J, et al. Muscle satellite cells and endothelial cells: close neighbors and privileged partners. Mol
Biol Cell. 2007;18:1397–409. CAS PubMed PubMed Central Google Scholar * Fang J, Feng C, Chen W, Hou P, Liu Z, Zuo M, et al. Redressing the interactions between stem cells and immune
system in tissue regeneration. Biol Direct. 2021;16:18. CAS PubMed PubMed Central Google Scholar * Seto N, Torres-Ruiz JJ, Carmona-Rivera C, Pinal-Fernandez I, Pak K, Purmalek MM, et al.
Neutrophil dysregulation is pathogenic in idiopathic inflammatory myopathies. JCI Insight. 2020;5:e134189. PubMed PubMed Central Google Scholar * Gao S, Zuo X, Liu D, Xiao Y, Zhu H,
Zhang H, et al. The roles of neutrophil serine proteinases in idiopathic inflammatory myopathies. Arthritis Res Ther. 2018;20:134. PubMed PubMed Central Google Scholar * Reimann J,
Schnell S, Schwartz S, Kappes-Horn K, Dodel R, Bacher M. Macrophage migration inhibitory factor in normal human skeletal muscle and inflammatory myopathies. J Neuropathol Exp Neurol.
2010;69:654–62. CAS PubMed Google Scholar * Preuße C, Goebel HH, Held J, Wengert O, Scheibe F, Irlbacher K, et al. Immune-mediated necrotizing myopathy is characterized by a specific
Th1-M1 polarized immune profile. Am J Pathol. 2012;181:2161–71. PubMed Google Scholar * Allenbach Y, Chaara W, Rosenzwajg M, Six A, Prevel N, Mingozzi F, et al. Th1 response and systemic
treg deficiency in inclusion body myositis. PLoS ONE. 2014;9:e88788. PubMed PubMed Central Google Scholar * Moran EM, Mastaglia FL. Cytokines in immune-mediated inflammatory myopathies:
cellular sources, multiple actions and therapeutic implications. Clin Exp Immunol. 2014;178:405–15. CAS PubMed PubMed Central Google Scholar * Greenberg SA, Higgs BW, Morehouse C, Walsh
RJ, Kong SW, Brohawn P, et al. Relationship between disease activity and type 1 interferon- and other cytokine-inducible gene expression in blood in dermatomyositis and polymyositis. Genes
Immun. 2012;13:207–13. CAS PubMed Google Scholar * Ladislau L, Suárez-Calvet X, Toquet S, Landon-Cardinal O, Amelin D, Depp M, et al. JAK inhibitor improves type I interferon induced
damage: proof of concept in dermatomyositis. Brain J Neurol. 2018;141:1609–21. Google Scholar * Chevrel G, Page G, Granet C, Streichenberger N, Varennes A, Miossec P. Interleukin-17
increases the effects of IL-1 beta on muscle cells: arguments for the role of T cells in the pathogenesis of myositis. J Neuroimmunol. 2003;137:125–33. CAS PubMed Google Scholar * Fréret
M, Drouot L, Obry A, Ahmed-Lacheheb S, Dauly C, Adriouch S, et al. Overexpression of MHC class I in muscle of lymphocyte-deficient mice causes a severe myopathy with induction of the
unfolded protein response. Am J Pathol. 2013;183:893–904. PubMed Google Scholar * Nagaraju K, Casciola-Rosen L, Lundberg I, Rawat R, Cutting S, Thapliyal R, et al. Activation of the
endoplasmic reticulum stress response in autoimmune myositis: potential role in muscle fiber damage and dysfunction. Arthritis Rheumatol. 2005;52:1824–35. CAS Google Scholar * Page G,
Chevrel G, Miossec P. Anatomic localization of immature and mature dendritic cell subsets in dermatomyositis and polymyositis: interaction with chemokines and Th1 cytokine-producing cells.
Arthritis Rheumatol. 2004;50:199–208. CAS Google Scholar * Wang S, Wang H, Feng C, Li C, Li Z, He J, et al. The regulatory role and therapeutic application of pyroptosis in musculoskeletal
diseases. Cell Death Discov. 2022;8:492. CAS PubMed PubMed Central Google Scholar * Salajegheh M, Pinkus JL, Amato AA, Morehouse C, Jallal B, Yao Y, et al. Permissive environment for
B-cell maturation in myositis muscle in the absence of B-cell follicles. Muscle Nerve. 2010;42:576–83. PubMed Google Scholar * Radke J, Koll R, Preuße C, Pehl D, Todorova K, Schönemann C,
et al. Architectural B-cell organization in skeletal muscle identifies subtypes of dermatomyositis. Neurol Neuroimmunol Neuroinflamm. 2018;5:e451. PubMed PubMed Central Google Scholar *
Stuhlmüller B, Schneider U, González-González J-B, Feist E. Disease specific autoantibodies in idiopathic inflammatory myopathies. Front Neurol. 2019;10:438. PubMed PubMed Central Google
Scholar * Arouche-Delaperche L, Allenbach Y, Amelin D, Preusse C, Mouly V, Mauhin W, et al. Pathogenic role of anti-signal recognition protein and anti-3-Hydroxy-3-methylglutaryl-CoA
reductase antibodies in necrotizing myopathies: myofiber atrophy and impairment of muscle regeneration in necrotizing autoimmune myopathies. Ann Neurol. 2017;81:538–48. CAS PubMed Google
Scholar * Bergua C, Chiavelli H, Allenbach Y, Arouche-Delaperche L, Arnoult C, Bourdenet G, et al. In vivo pathogenicity of IgG from patients with anti-SRP or anti-HMGCR autoantibodies in
immune-mediated necrotising myopathy. Ann Rheum Dis. 2019;78:131–9. CAS PubMed Google Scholar * Fujiyama T, Ito T, Ogawa N, Suda T, Tokura Y, Hashizume H. Preferential infiltration of
interleukin-4-producing CXCR4+ T cells in the lesional muscle but not skin of patients with dermatomyositis. Clin Exp Immunol. 2014;177:110–20. CAS PubMed PubMed Central Google Scholar *
Rostasy KM, Schmidt J, Bahn E, Pfander T, Piepkorn M, Wilichowski E, et al. Distinct inflammatory properties of late-activated macrophages in inflammatory myopathies. Acta Myol.
2008;27:49–53. CAS PubMed PubMed Central Google Scholar * Noda S, Koike H, Maeshima S, Nakanishi H, Iijima M, Matsuo K, et al. Transforming growth factor-β signaling is upregulated in
sporadic inclusion body myositis. Muscle Nerve. 2017;55:741–7. CAS PubMed Google Scholar * Burks TN, Cohn RD. Role of TGF-β signaling in inherited and acquired myopathies. Skelet Muscle.
2011;1:19. CAS PubMed PubMed Central Google Scholar * Confalonieri P, Bernasconi P, Cornelio F, Mantegazza R. Transforming growth factor-beta 1 in polymyositis and dermatomyositis
correlates with fibrosis but not with mononuclear cell infiltrate. J Neuropathol Exp Neurol. 1997;56:479–84. CAS PubMed Google Scholar * Girardi F, Taleb A, Ebrahimi M, Datye A, Gamage
DG, Peccate C, et al. TGFβ signaling curbs cell fusion and muscle regeneration. Nat Commun. 2021;12:750. CAS PubMed PubMed Central Google Scholar * Uezumi A, Ito T, Morikawa D, Shimizu
N, Yoneda T, Segawa M, et al. Fibrosis and adipogenesis originate from a common mesenchymal progenitor in skeletal muscle. J Cell Sci. 2011;124:3654–64. CAS PubMed Google Scholar * Saito
Y, Chikenji TS, Matsumura T, Nakano M, Fujimiya M. Exercise enhances skeletal muscle regeneration by promoting senescence in fibro-adipogenic progenitors. Nat Commun. 2020;11:889. CAS
PubMed PubMed Central Google Scholar * Wanschitz JV, Dubourg O, Lacene E, Fischer MB, Höftberger R, Budka H, et al. Expression of myogenic regulatory factors and myo-endothelial
remodeling in sporadic inclusion body myositis. Neuromuscul Disord. 2013;23:75–83. PubMed PubMed Central Google Scholar * Schiaffino S, Pereira MG, Ciciliot S, Rovere-Querini P.
Regulatory T cells and skeletal muscle regeneration. FEBS J. 2017;284:517–24. CAS PubMed Google Scholar * Henriques-Pons A, Nagaraju K. Nonimmune mechanisms of muscle damage in myositis:
role of the endoplasmic reticulum stress response and autophagy in the disease pathogenesis. Curr Opin Rheumatol. 2009;21:581–7. PubMed PubMed Central Google Scholar * Benveniste O,
Stenzel W, Hilton-Jones D, Sandri M, Boyer O, van Engelen BGM. Amyloid deposits and inflammatory infiltrates in sporadic inclusion body myositis: the inflammatory egg comes before the
degenerative chicken. Acta Neuropathol. 2015;129:611–24. CAS PubMed PubMed Central Google Scholar * Loell I, Lundberg IE. Can muscle regeneration fail in chronic inflammation: a weakness
in inflammatory myopathies? J Intern Med. 2011;269:243–57. CAS PubMed Google Scholar * Morosetti R, Broccolini A, Sancricca C, Gliubizzi C, Gidaro T, Tonali PA, et al. Increased aging in
primary muscle cultures of sporadic inclusion-body myositis. Neurobiol Aging. 2010;31:1205–14. PubMed Google Scholar * Barsotti S, Lundberg IE. Current treatment for myositis. Curr Treat
Options Rheumatol. 2018;4:299–315. Google Scholar * Schakman O, Kalista S, Barbé C, Loumaye A, Thissen JP. Glucocorticoid-induced skeletal muscle atrophy. Int J Biochem Cell Biol.
2013;45:2163–72. CAS PubMed Google Scholar * Ponticelli C, Glassock RJ. Prevention of complications from use of conventional immunosuppressants: a critical review. J Nephrol.
2019;32:851–70. PubMed Google Scholar * Khoo T, Limaye V. Biologic therapy in the idiopathic inflammatory myopathies. Rheumatol Int. 2020;40:191–205. PubMed Google Scholar * Paik JJ,
Casciola-Rosen L, Shin JY, Albayda J, Tiniakou E, Leung DG, et al. Study of tofacitinib in refractory dermatomyositis (STIR): an open label pilot study of 10 patients. Arthritis Rheumatol.
2020. https://doi.org/10.1002/art.41602. * Julien S, Vadysirisack D, Sayegh C, Ragunathan S, Tang Y, Briand E, et al. Prevention of anti-HMGCR immune-mediated necrotising myopathy by C5
complement inhibition in a humanised mouse model. Biomedicines. 2022;10:2036. CAS PubMed PubMed Central Google Scholar * Mammen AL, Amato AA, Dimachkie MM, Chinoy H, Hussain Y, Lilleker
JB, et al. Zilucoplan in immune-mediated necrotising myopathy: a phase 2, randomised, double-blind, placebo-controlled, multicentre trial. Lancet Rheumatol. 2023;5:e67–76. CAS PubMed
Google Scholar * Schmidt K, Schmidt J. Inclusion body myositis: advancements in diagnosis, pathomechanisms, and treatment. Curr Opin Rheumatol. 2017;29:632–8. PubMed Google Scholar * Dao
T, Green AE, Kim YA, Bae S-J, Ha K-T, Gariani K, et al. Sarcopenia and muscle aging: a brief overview. Endocrinol Metab. 2020;35:716–32. CAS Google Scholar * Bohan A, Peter JB.
Polymyositis and dermatomyositis (second of two parts). N Engl J Med. 1975;292:403–7. CAS PubMed Google Scholar * Wang D, Zhang H, Cao M, Tang Y, Liang J, Feng X, et al. Efficacy of
allogeneic mesenchymal stem cell transplantation in patients with drug-resistant polymyositis and dermatomyositis. Ann Rheum Dis. 2011;70:1285–8. PubMed Google Scholar * Lai Q, Yu L, Qiu
Y, Chen L, Huang J, Li Y, et al. Umbilical cord blood mesenchymal stem cells transplantion for polymyositis/dermatomyositis:variation of Th cytokines. Chin J Tissue Eng Res. 2015;14:2186–91.
Google Scholar * Ra JC, Kang SK, Shin IS, Park HG, Joo SA, Kim JG, et al. Stem cell treatment for patients with autoimmune disease by systemic infusion of culture-expanded autologous
adipose tissue derived mesenchymal stem cells. J Transl Med. 2011;9:181. PubMed PubMed Central Google Scholar * Senesi L, De Francesco F, Farinelli L, Manzotti S, Gagliardi G, Papalia GF,
et al. Mechanical and enzymatic procedures to isolate the stromal vascular fraction from adipose tissue: preliminary results. Front Cell Dev Biol. 2019;7:88. PubMed PubMed Central Google
Scholar * Condé-Green A, Kotamarti VS, Sherman LS, Keith JD, Lee ES, Granick MS, et al. Shift toward mechanical isolation of adipose-derived stromal vascular fraction: review of upcoming
techniques. Plast Reconstr Surg Glob Open. 2016;4:e1017. PubMed PubMed Central Google Scholar * van Dongen JA, Tuin AJ, Spiekman M, Jansma J, van der Lei B, Harmsen MC. Comparison of
intraoperative procedures for isolation of clinical grade stromal vascular fraction for regenerative purposes: a systematic review. J Tissue Eng Regen Med. 2018;12:e261–74. PubMed Google
Scholar * Yao Y, Dong Z, Liao Y, Zhang P, Ma J, Gao J, et al. Adipose extracellular matrix/stromal vascular fraction gel: a novel adipose tissue-derived injectable for stem cell therapy.
Plast Reconstr Surg. 2017;139:867–79. CAS PubMed Google Scholar * Laschke MW, Menger MD. Adipose tissue-derived microvascular fragments: natural vascularization units for regenerative
medicine. Trends Biotechnol. 2015;33:442–8. CAS PubMed Google Scholar * Toyserkani NM, Jørgensen MG, Tabatabaeifar S, Jensen CH, Sheikh SP, Sørensen JA. Concise review: a safety
assessment of adipose-derived cell therapy in clinical trials: a systematic review of reported adverse events. Stem Cells Transl Med. 2017;6:1786–94. PubMed PubMed Central Google Scholar
* Murdoch B, Zarzeczny A, Caulfield T. Exploiting science? A systematic analysis of complementary and alternative medicine clinic websites’ marketing of stem cell therapies. BMJ Open.
2018;8:e019414. PubMed PubMed Central Google Scholar * Knoepfler PS. Rapid change of a cohort of 570 unproven stem cell clinics in the USA over 3 years. Regen Med. 2019;14:735–40. CAS
PubMed Google Scholar * Commissioner, O of the. Innovative regenerative medicine therapies – patient safety comes first. FDA_._ 2021.
https://www.fda.gov/news-events/fda-voices/innovative-regenerative-medicine-therapies-patient-safety-comes-first. * Andia I, Maffulli N, Burgos-Alonso N. Stromal vascular fraction
technologies and clinical applications. Expert Opin Biol Ther. 2019;19:1289–305. PubMed Google Scholar * Bauer G, Elsallab M, Abou-El-Enein M. Concise review: a comprehensive analysis of
reported adverse events in patients receiving unproven stem cell-based interventions. Stem Cells Transl Med. 2018;7:676–85. PubMed PubMed Central Google Scholar * Pritchett JW. The debit
side of stem-cell joint injections: a prospective cohort study. Curr Orthop Pract. 2021;32:118–23. Google Scholar * Ge J, Guo L, Wang S, Zhang Y, Cai T, Zhao RCH, et al. The size of
mesenchymal stem cells is a significant cause of vascular obstructions and stroke. Stem Cell Rev Rep. 2014;10:295–303. CAS PubMed Google Scholar * Monreal J. Functional and aesthetic
recovery of congenital muscular torticollis with intramuscular stromal vascular fraction enriched fat grafting. Cureus. 2017;9:e975. PubMed PubMed Central Google Scholar * Lee H-B, Park
S-W, Kim J, Hwang K-C, Kim I-K, Sun H-M, et al. Intramuscular injection of adipose tissue derived stromal vascular fraction in subjects with poliomyelitis: case reports. Int J Stem Cell Res
Ther. 2019;1:20–27. Google Scholar * Bura A, Planat-Benard V, Bourin P, Silvestre J-S, Gross F, Grolleau J-L, et al. Phase I trial: the use of autologous cultured adipose-derived
stroma/stem cells to treat patients with non-revascularizable critical limb ischemia. Cytotherapy. 2014;16:245–57. CAS PubMed Google Scholar * Lee HC, An SG, Lee HW, Park J-S, Cha KS,
Hong TJ, et al. Safety and effect of adipose tissue-derived stem cell implantation in patients with critical limb ischemia: a pilot study. Circ J J Jpn Circ Soc. 2012;76:1750–60. CAS Google
Scholar * Hamidian Jahromi S, Davies JE. Concise review: skeletal muscle as a delivery route for mesenchymal stromal cells. Stem Cells Transl Med. 2019;8:456–65. PubMed PubMed Central
Google Scholar * Braid LR, Wood CA, Wiese DM, Ford BN. Intramuscular administration potentiates extended dwell time of mesenchymal stromal cells compared to other routes. Cytotherapy.
2018;20:232–44. PubMed Google Scholar * Hamidian Jahromi S, Estrada C, Li Y, Cheng E, Davies JE. Human umbilical cord perivascular cells and human bone marrow mesenchymal stromal cells
transplanted intramuscularly respond to a distant source of inflammation. Stem Cells Dev. 2018;27:415–29. CAS PubMed Google Scholar * Puissant B, Barreau C, Bourin P, Clavel C, Corre J,
Bousquet C, et al. Immunomodulatory effect of human adipose tissue-derived adult stem cells: comparison with bone marrow mesenchymal stem cells. Br J Haematol. 2005;129:118–29. PubMed
Google Scholar * Najar M, Raicevic G, Fayyad-Kazan H, De Bruyn C, Bron D, Toungouz M, et al. Impact of different mesenchymal stromal cell types on T-cell activation, proliferation and
migration. Int Immunopharmacol. 2013;15:693–702. CAS PubMed Google Scholar * Ketterl N, Brachtl G, Schuh C, Bieback K, Schallmoser K, Reinisch A, et al. A robust potency assay highlights
significant donor variation of human mesenchymal stem/progenitor cell immune modulatory capacity and extended radio-resistance. Stem Cell Res Ther. 2015;6:236. PubMed PubMed Central Google
Scholar * Bowles AC, Strong AL, Wise RM, Thomas RC, Gerstein BY, Dutreil MF, et al. Adipose stromal vascular fraction-mediated improvements at late-stage disease in a murine model of
multiple sclerosis. Stem Cells. 2017;35:532–44. CAS PubMed Google Scholar * Cui L, Yin S, Liu W, Li N, Zhang W, Cao Y. Expanded adipose-derived stem cells suppress mixed lymphocyte
reaction by secretion of prostaglandin E2. Tissue Eng. 2007;13:1185–95. CAS PubMed Google Scholar * DelaRosa O, Lombardo E, Beraza A, Mancheño-Corvo P, Ramirez C, Menta R, et al.
Requirement of IFN-gamma-mediated indoleamine 2,3-dioxygenase expression in the modulation of lymphocyte proliferation by human adipose-derived stem cells. Tissue Eng Part A.
2009;15:2795–806. CAS PubMed Google Scholar * Menta R, Mancheño-Corvo P, Del Río B, Ramírez C, DelaRosa O, Dalemans W, et al. Tryptophan concentration is the main mediator of the capacity
of adipose mesenchymal stromal cells to inhibit T-lymphocyte proliferation in vitro. Cytotherapy. 2014;16:1679–91. CAS PubMed Google Scholar * Najar M, Raicevic G, Fayyad-Kazan H, De
Bruyn C, Bron D, Toungouz M, et al. Bone marrow mesenchymal stromal cells induce proliferative, cytokinic and molecular changes during the T cell response: the importance of the IL-10/CD210
axis. Stem Cell Rev Rep. 2015;11:442–52. CAS PubMed Google Scholar * Cho K-S, Park M-K, Kang S-A, Park H-Y, Hong S-L, Park H-K, et al. Adipose-derived stem cells ameliorate allergic
airway inflammation by inducing regulatory T cells in a mouse model of asthma. Mediators Inflamm. 2014;2014:436476. PubMed PubMed Central Google Scholar * Zeng Q, Sun X, Xiao L, Xie Z,
Bettini M, Deng T. A unique population: adipose-resident regulatory T cells. Front Immunol. 2018;9:2075. PubMed PubMed Central Google Scholar * Németh K, Leelahavanichkul A, Yuen PST,
Mayer B, Parmelee A, Doi K, et al. Bone marrow stromal cells attenuate sepsis via prostaglandin E(2)-dependent reprogramming of host macrophages to increase their interleukin-10 production.
Nat Med. 2009;15:42–9. PubMed Google Scholar * Shi J, Lu P, Shen W, He R, Yang M-W, Fang Y, et al. CD90 highly expressed population harbors a stemness signature and creates an
immunosuppressive niche in pancreatic cancer. Cancer Lett. 2019;453:158–69. PubMed Google Scholar * Ménard C, Dulong J, Roulois D, Hébraud B, Verdière L, Pangault C, et al. Integrated
transcriptomic, phenotypic, and functional study reveals tissue-specific immune properties of mesenchymal stromal cells. Stem Cells. 2020;38:146–59. PubMed Google Scholar * Espagnolle N,
Balguerie A, Arnaud E, Sensebé L, Varin A. CD54-mediated interaction with pro-inflammatory macrophages increases the immunosuppressive function of human mesenchymal stromal cells. Stem Cell
Rep. 2017;8:961–76. CAS Google Scholar * Lumeng CN, Bodzin JL, Saltiel AR. Obesity induces a phenotypic switch in adipose tissue macrophage polarization. J Clin Invest. 2007;117:175–84.
CAS PubMed PubMed Central Google Scholar * Williams L, Bradley L, Smith A, Foxwell B. Signal transducer and activator of transcription 3 is the dominant mediator of the anti-inflammatory
effects of IL-10 in human macrophages. J Immunol. 2004;172:567–76. CAS PubMed Google Scholar * Hutchins AP, Diez D, Miranda-Saavedra D. The IL-10/STAT3-mediated anti-inflammatory
response: recent developments and future challenges. Brief Funct Genom. 2013;12:489–98. CAS Google Scholar * Murray PJ. The primary mechanism of the IL-10-regulated antiinflammatory
response is to selectively inhibit transcription. Proc Natl Acad Sci USA. 2005;102:8686–91. CAS PubMed PubMed Central Google Scholar * Sato Y, Ohshima T, Kondo T. Regulatory role of
endogenous interleukin-10 in cutaneous inflammatory response of murine wound healing. Biochem Biophys Res Commun. 1999;265:194–9. CAS PubMed Google Scholar * Shi J-H, Guan H, Shi S, Cai
W-X, Bai X-Z, Hu X-L, et al. Protection against TGF-β1-induced fibrosis effects of IL-10 on dermal fibroblasts and its potential therapeutics for the reduction of skin scarring. Arch
Dermatol Res. 2013;305:341–52. CAS PubMed Google Scholar * Shi J, Li J, Guan H, Cai W, Bai X, Fang X, et al. Anti-fibrotic actions of interleukin-10 against hypertrophic scarring by
activation of PI3K/AKT and STAT3 signaling pathways in scar-forming fibroblasts. PLoS ONE. 2014;9:e98228. PubMed PubMed Central Google Scholar * Serratrice N, Bruzzese L, Magalon J, Véran
J, Giraudo L, Aboudou H, et al. New fat-derived products for treating skin-induced lesions of scleroderma in nude mice. Stem Cell Res Ther. 2014;5:138. PubMed PubMed Central Google
Scholar * Mattei A, Magalon J, Bertrand B, Grimaud F, Revis J, Velier M, et al. Autologous adipose-derived stromal vascular fraction and scarred vocal folds: first clinical case report.
Stem Cell Res Ther. 2018;9:202. PubMed PubMed Central Google Scholar * Domergue S, Bony C, Maumus M, Toupet K, Frouin E, Rigau V, et al. Comparison between stromal vascular fraction and
adipose mesenchymal stem cells in remodeling hypertrophic scars. PLoS ONE. 2016;11:e0156161. PubMed PubMed Central Google Scholar * Usunier B, Benderitter M, Tamarat R, Chapel A.
Management of fibrosis: the mesenchymal stromal cells breakthrough. Stem Cells Int. 2014;2014:340257. PubMed PubMed Central Google Scholar * Burbridge MF, Cogé F, Galizzi JP, Boutin JA,
West DC, Tucker GC. The role of the matrix metalloproteinases during in vitro vessel formation. Angiogenesis. 2002;5:215–26. CAS PubMed Google Scholar * Jin E, Chae D-S, Son M, Kim S-W.
Angiogenic characteristics of human stromal vascular fraction in ischemic hindlimb. Int J Cardiol. 2017;234:38–47. PubMed Google Scholar * Planat-Benard V, Silvestre J-S, Cousin B, André
M, Nibbelink M, Tamarat R, et al. Plasticity of human adipose lineage cells toward endothelial cells: physiological and therapeutic perspectives. Circulation. 2004;109:656–63. PubMed Google
Scholar * Traktuev DO, Merfeld-Clauss S, Li J, Kolonin M, Arap W, Pasqualini R, et al. A population of multipotent CD34-positive adipose stromal cells share pericyte and mesenchymal
surface markers, reside in a periendothelial location, and stabilize endothelial networks. Circ Res. 2008;102:77–85. CAS PubMed Google Scholar * Traktuev DO, Prater DN, Merfeld-Clauss S,
Sanjeevaiah AR, Saadatzadeh MR, Murphy M, et al. Robust functional vascular network formation in vivo by cooperation of adipose progenitor and endothelial cells. Circ Res. 2009;104:1410–20.
CAS PubMed Google Scholar * Hellström M, Kalén M, Lindahl P, Abramsson A, Betsholtz C. Role of PDGF-B and PDGFR-beta in recruitment of vascular smooth muscle cells and pericytes during
embryonic blood vessel formation in the mouse. Development. 1999;126:3047–55. PubMed Google Scholar * Lopatina T, Bruno S, Tetta C, Kalinina N, Porta M, Camussi G. Platelet-derived growth
factor regulates the secretion of extracellular vesicles by adipose mesenchymal stem cells and enhances their angiogenic potential. Cell Commun Signal. 2014;12:26. PubMed PubMed Central
Google Scholar * Zhou L, Xia J, Qiu X, Wang P, Jia R, Chen Y, et al. In vitro evaluation of endothelial progenitor cells from adipose tissue as potential angiogenic cell sources for bladder
angiogenesis. PLoS ONE. 2015;10:e0117644. PubMed PubMed Central Google Scholar * Matsui J, Wakabayashi T, Asada M, Yoshimatsu K, Okada M. Stem cell factor/c-kit signaling promotes the
survival, migration, and capillary tube formation of human umbilical vein endothelial cells. J Biol Chem. 2004;279:18600–7. CAS PubMed Google Scholar * Stapor PC, Sweat RS, Dashti DC,
Betancourt AM, Murfee WL. Pericyte dynamics during angiogenesis: new insights from new identities. J Vasc Res. 2014;51:163–74. PubMed Google Scholar * Lindahl P, Johansson BR, Levéen P,
Betsholtz C. Pericyte loss and microaneurysm formation in PDGF-B-deficient mice. Science. 1997;277:242–5. CAS PubMed Google Scholar * Ascenzi F, Barberi L, Dobrowolny G, Villa Nova
Bacurau A, Nicoletti C, Rizzuto E, et al. Effects of IGF-1 isoforms on muscle growth and sarcopenia. Aging Cell. 2019;18:e12954. PubMed PubMed Central Google Scholar * Lee J-H, Kemp DM.
Human adipose-derived stem cells display myogenic potential and perturbed function in hypoxic conditions. Biochem Biophys Res Commun. 2006;341:882–8. CAS PubMed Google Scholar * Mizuno H,
Zuk PA, Zhu M, Lorenz HP, Benhaim P, Hedrick MH. Myogenic differentiation by human processed lipoaspirate cells. Plast Reconstr Surg .2002;109:199–209. PubMed Google Scholar * Goudenege
S, Pisani DF, Wdziekonski B, Di Santo JP, Bagnis C, Dani C, et al. Enhancement of myogenic and muscle repair capacities of human adipose-derived stem cells with forced expression of MyoD.
Mol Ther J Am Soc Gene Ther. 2009;17:1064–72. CAS Google Scholar * Di Rocco G, Iachininoto MG, Tritarelli A, Straino S, Zacheo A, Germani A, et al. Myogenic potential of
adipose-tissue-derived cells. J Cell Sci. 2006;119:2945–52. PubMed Google Scholar * Bacou F, Andalousi RBE, Daussin P-A, Micallef J-P, Levin JM, Chammas M, et al. Transplantation of
adipose tissue-derived stromal cells increases mass and functional capacity of damaged skeletal muscle. Cell Transpl. 2004;13:103–11. Google Scholar * Zimowska M, Archacka K, Brzoska E, Bem
J, Czerwinska AM, Grabowska I, et al. IL-4 and SDF-1 increase adipose tissue-derived stromal cell ability to improve rat skeletal muscle regeneration. Int J Mol Sci. 2020;21:3302. CAS
PubMed PubMed Central Google Scholar * Rodriguez A-M, Pisani D, Dechesne CA, Turc-Carel C, Kurzenne J-Y, Wdziekonski B, et al. Transplantation of a multipotent cell population from human
adipose tissue induces dystrophin expression in the immunocompetent mdx mouse. J Exp Med. 2005;201:1397–405. CAS PubMed PubMed Central Google Scholar Download references ACKNOWLEDGEMENTS
This work was supported by the Fondation pour la Recherche Médicale (grant # FDM201806006523). The authors are grateful to Nikki Sabourin-Gibbs, Rouen University Hospital, for her help in
editing the manuscript. AUTHOR INFORMATION Author notes * These authors contributed equally: S. Gandolfi, B. Pileyre. AUTHORS AND AFFILIATIONS * Univ Rouen Normandie, INSERM U1234, FOCIS
Center of Excellence PAn’THER, F-76000, Rouen, France S. Gandolfi, B. Pileyre, L. Drouot & I. Dubus * Toulouse University Hospital, Department of Plastic and Reconstructive Surgery,
F-31000, Toulouse, France S. Gandolfi * Centre Henri Becquerel, Department of Pharmacy, F-76000, Rouen, France B. Pileyre * Univ Rouen Normandie, INSERM U1234, FOCIS Center of Excellence
PAn’THER, CHU Rouen, Department of Plastic, Reconstructive and Hand Surgery, F-76000, Rouen, France I. Auquit-Auckbur * Univ Rouen Normandie, INSERM U1234, FOCIS Center of Excellence
PAn’THER, CHU Rouen, Department of Immunology and Biotherapy, F-76000, Rouen, France J. Martinet Authors * S. Gandolfi View author publications You can also search for this author inPubMed
Google Scholar * B. Pileyre View author publications You can also search for this author inPubMed Google Scholar * L. Drouot View author publications You can also search for this author
inPubMed Google Scholar * I. Dubus View author publications You can also search for this author inPubMed Google Scholar * I. Auquit-Auckbur View author publications You can also search for
this author inPubMed Google Scholar * J. Martinet View author publications You can also search for this author inPubMed Google Scholar CONTRIBUTIONS SG and BP were the manuscript’s principal
authors. LD, ID, IA, and JM participated in manuscript writing and editing. All authors approved the final manuscript. CORRESPONDING AUTHOR Correspondence to B. Pileyre. ETHICS DECLARATIONS
COMPETING INTERESTS The authors declare no competing interests. ADDITIONAL INFORMATION PUBLISHER’S NOTE Springer Nature remains neutral with regard to jurisdictional claims in published
maps and institutional affiliations. RIGHTS AND PERMISSIONS OPEN ACCESS This article is licensed under a Creative Commons Attribution 4.0 International License, which permits use, sharing,
adaptation, distribution and reproduction in any medium or format, as long as you give appropriate credit to the original author(s) and the source, provide a link to the Creative Commons
license, and indicate if changes were made. The images or other third party material in this article are included in the article’s Creative Commons license, unless indicated otherwise in a
credit line to the material. If material is not included in the article’s Creative Commons license and your intended use is not permitted by statutory regulation or exceeds the permitted
use, you will need to obtain permission directly from the copyright holder. To view a copy of this license, visit http://creativecommons.org/licenses/by/4.0/. Reprints and permissions ABOUT
THIS ARTICLE CITE THIS ARTICLE Gandolfi, S., Pileyre, B., Drouot, L. _et al._ Stromal vascular fraction in the treatment of myositis. _Cell Death Discov._ 9, 346 (2023).
https://doi.org/10.1038/s41420-023-01605-9 Download citation * Received: 13 March 2023 * Revised: 01 August 2023 * Accepted: 14 August 2023 * Published: 19 September 2023 * DOI:
https://doi.org/10.1038/s41420-023-01605-9 SHARE THIS ARTICLE Anyone you share the following link with will be able to read this content: Get shareable link Sorry, a shareable link is not
currently available for this article. Copy to clipboard Provided by the Springer Nature SharedIt content-sharing initiative