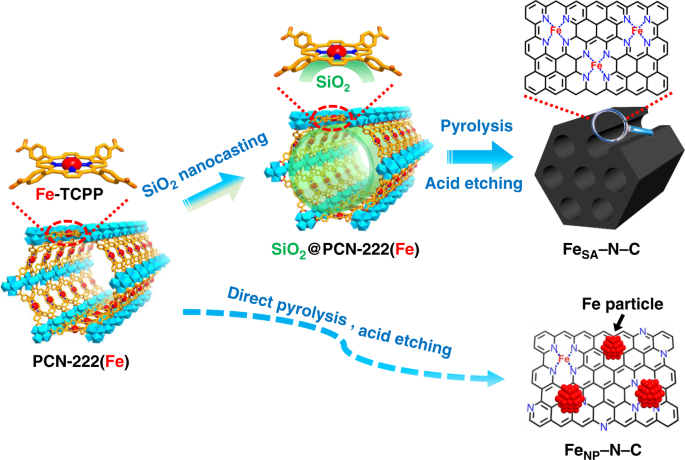
Nanocasting sio2 into metal–organic frameworks imparts dual protection to high-loading fe single-atom electrocatalysts
- Select a language for the TTS:
- UK English Female
- UK English Male
- US English Female
- US English Male
- Australian Female
- Australian Male
- Language selected: (auto detect) - EN
Play all audios:

ABSTRACT Single-atom catalysts (SACs) have sparked broad interest recently while the low metal loading poses a big challenge for further applications. Herein, a dual protection strategy has
been developed to give high-content SACs by nanocasting SiO2 into porphyrinic metal–organic frameworks (MOFs). The pyrolysis of SiO2@MOF composite affords single-atom Fe implanted N-doped
porous carbon (FeSA–N–C) with high Fe loading (3.46 wt%). The spatial isolation of Fe atoms centered in porphyrin linkers of MOF sets the first protective barrier to inhibit the Fe
agglomeration during pyrolysis. The SiO2 in MOF provides additional protection by creating thermally stable FeN4/SiO2 interfaces. Thanks to the high-density FeSA sites, FeSA–N–C demonstrates
excellent oxygen reduction performance in both alkaline and acidic medias. Meanwhile, FeSA–N–C also exhibits encouraging performance in proton exchange membrane fuel cell, demonstrating
great potential for practical application. More far-reaching, this work grants a general synthetic methodology toward high-content SACs (such as FeSA, CoSA, NiSA). SIMILAR CONTENT BEING
VIEWED BY OTHERS OPTIMIZING CHARGE PATHWAYS BY INTERFACE ENGINEERING IN FE2O3/CO3O4/CO(PO3)2 HETEROSTRUCTURES FOR SUPERIOR OXYGEN EVOLUTION REACTION Article Open access 27 February 2025
IMPACT OF IONOMERS ON POROUS FE-N-C CATALYSTS FOR ALKALINE OXYGEN REDUCTION IN GAS DIFFUSION ELECTRODES Article Open access 31 January 2025 DUAL-SITE SEGMENTALLY SYNERGISTIC CATALYSIS
MECHANISM: BOOSTING COFES_X_ NANOCLUSTER FOR SUSTAINABLE WATER OXIDATION Article Open access 26 February 2024 INTRODUCTION Single-atom catalysts (SACs), with the maximal utilization of metal
atoms, open up a new frontier and attract increasing attention in catalysis1,2,3,4,5,6,7,8,9,10. Integrated with plenty of merits, including highly dispersed sites, high activity, excellent
selectivity, and good reusability, SACs have been regarded as an ideal platform to bridge the gap between homogenous and heterogeneous catalysts1,2,3,4. Nevertheless, isolated metal atoms
in SACs tend to agglomerate due to the high surface energy. Though significant progress has been achieved to ensure the atomic dispersion of metal atoms, metal loadings of SACs are basically
low (<1 wt%). The construction methodology of stable SACs, especially in high metal loadings, is highly desired yet remains a grand challenge11,12,13. In addition, to boost the catalytic
performance of SACs, their physical features, including porous structure and surface area, which dominate the accessibility to active sites, should also be optimized14,15,16. Metal–organic
frameworks (MOFs)17,18,19,20,21,22,23,24,25, featuring well-defined and tailored structures, present particular advantages in the precise fabrication of catalysts, especially
SACs26,27,28,29,30,31,32,33. The present synthetic strategy for MOF-based SACs is to augment the distance between adjacent metal atoms based on the mixed metal/ligand and pore confinement,
which effectively inhibits the agglomeration of metal atoms under pyrolysis27,28,29,30. Unfortunately, these strategies cause the decrease of metal loadings. As a result, even bearing the
structural advantages in MOF-based SACs, their metal loadings are unsatisfying, such as FeSA (usually, <2 wt%)26,27,28,29,30,31,32,33. In addition, most of reported MOF-derived SACs
possess micropores (<2 nm), which is unfavorable to mass transfer in catalytic process27,30. To address these issues, alternative synthetic strategies for MOF-derived SACs are imperative
to improving the metal loadings and pore structures. To realize SACs with high metal loadings, the main obstacle to overcome is their easy-to-migrate feature due to their high surface
energy, especially under pyrolysis. It was found that inorganic silica (SiO2) protection method can stabilize metal nanoparticles/clusters by decreasing surface energy of metal
atoms1,34,35,36,37,38, which might be effective toward the stabilization of highly loaded SACs. Making full use of the porosity of MOFs, SiO2 can be easily nanocasted into the pore space of
MOFs to interact with the isolated metal atoms on MOF skeleton, which would significantly lower their surface energy. In consequence, the introduction of SiO2 into MOFs, when integrated with
the merits of MOFs, should be a very promising route to improve metal loadings in SACs. In this work, we creatively put forward a nanocasting strategy to introduce SiO2 into the mesopores
of a porphyrinic MOF, PCN-222(Fe), featuring single Fe(III) site in each porphyrin linker39,40,41. Thanks to the 1D mesochannel with a diameter of ~3.2 nm, SiO2 can be sufficiently filled
into PCN-222(Fe), forming thermal stable FeN4/SiO2 interfaces. Upon high-temperature pyrolysis and SiO2 removal, the single-atom Fe catalyst, denoted FeSA–N–C, with a Fe loading as high as
3.46 wt%, is obtained (Fig. 1). During the pyrolysis, the spatial isolation of Fe atoms anchored by N atoms in porphyrin linkers is the first protective barrier to inhibit the Fe
agglomeration. The silica in MOF channels serves as oxide substrate to interact with Fe atoms that can increase migration energy barrier of Fe atoms and prevent their aggregation. Meanwhile,
upon removal of silica, the porosity and surface area of the resultant N-doped porous carbon can be improved, benefiting the exposure of active sites and mass transfer. As a result, the
optimized FeSA–N–C exhibits excellent oxygen reduction reaction (ORR) performance, surpassing the state-of-the-art Pt/C, and almost all reported non-noble-metal catalysts, in both alkaline
and the more challenging acidic solutions. Significantly, the FeSA–N–C reaches a current density of 292 mA cm−2 at 0.8 V and the highest power density of 0.68 W cm−2, comparable to that of
the best non-noble metal catalysts, in H2–O2 proton exchange membrane fuel cell (PEMFC). RESULTS SYNTHESIS AND CHARACTERIZATION OF FESA–N–C The PCN-222(Fe) was prepared by employing
trifluoroacetic acid (TFA), instead of the traditionally used benzoic acid, as a modulator (Supplementary Fig. 1)39. The scanning electron microscopy (SEM) image of PCN-222(Fe) presents the
uniform spindle morphology with a diameter ~250 nm (Fig. 2a). The low boiling point of TFA makes it easy to be removed by direct degassing without additional pre-activation process
(necessary for benzoic acid modulator) to deliver available pore space in PCN-222(Fe) (Supplementary Table 1). The N2 sorption with a typical type-IV isotherms present a high surface area up
to 2040 m2 g−1 and the pore size distribution suggests the mesochannels centered at 3.2 nm, in good agreement with the transmission electron microscopy (TEM) observation (Fig. 2b,
Supplementary Fig. 2). This greatly facilitates the subsequent introduction of tetraethylorthosilicate (TEOS) into PCN-222(Fe) mesopores for SiO2 nanocasting, after degassing the MOF at 150
°C. The facile TFA removal and mesoporosity guarantee the sufficient percolation of TEOS through the entire inner space of PCN-222(Fe). Upon HCl vapor treatment, TEOS in PCN-222(Fe) can be
hydrolyzed and condensed to silica, affording SiO2@PCN-222(Fe) composite with well-retained MOF crystallinity, thanks to the ultrahigh acidic stability of the MOF (Supplementary Fig. 1)39.
The infrared peak at 1090 cm−1 assignable to Si-O-Si clearly confirms the SiO2 formation (Supplementary Fig. 3)37. The reduced surface area (1190 m2 g–1) and mesopore size (2.9 nm) in the
composite, in reference to the parent MOF (BET of 2040 m2 g−1; pore size of 3.2 nm), further indicate the successful infiltration of SiO2 in PCN-222(Fe). The elemental mapping images of
SiO2@PCN-222(Fe) further illustrate the homogeneous dispersion of Si in PCN-222(Fe) (Supplementary Fig. 4). After the pyrolysis of SiO2@PCN-222(Fe) at 800 °C, the composite of metal (oxides)
stabilized by porous carbon is produced. Upon the removal of the oxide by acid etching, FeSA–N–C with retained spindle morphology is finally obtained and no particles are observed in TEM
images (Fig. 2c–e, Supplementary Fig. 5a). The elemental mapping images clearly demonstrate the homogenous dispersion of Fe and N elements on FeSA–N–C (Supplementary Fig. 6).
Aberration-corrected high-angle annular darkfield scanning transmission electron microscope (HAADF-STEM) observation shows the isolated and high-density bright spots, implying the formation
of single metal atoms (Fig. 2f, Supplementary Fig. 5b). The accurate contents of Fe (3.46 wt%) and N (4.87 wt%) have been quantified by inductively coupled plasma atomic emission
spectrometry (ICP) and elemental analysis, demonstrating the presence of single Fe atom and suggesting the higher Fe loading than almost all reported single-atom Fe-incorporated carbon-based
materials (Supplementary Table 2). Moreover, the quantitative analysis of X-ray photoelectron spectroscopy (XPS) and energy-dispersive spectroscopy further confirm the high loading of Fe,
in accordance with the ICP results (Supplementary Table 3). In addition, the content of Zr is extremely low, illustrating Fe is the dominated metal species in FeSA–N–C (Supplementary Table
3). As illustrated above, the perfect combination of PCN-222(Fe) and SiO2 can exert their respective advantages for the creation of the resultant FeSA–N–C, which are visually summarized
(Supplementary Fig. 7). Powder X-ray diffraction (PXRD) pattern of FeSA–N–C gives two broad peaks in the ranges of 20–30° and 40–45°, corresponding to (002) and (101) planes of graphitized
carbon, and no diffraction of Fe-based species is identifiable, in accordance with the TEM observation results (Fig. 2c–e, Supplementary Fig. 8). Raman scattering spectrum for FeSA–N–C gives
low intensity ratio (_I_D/_I_G = 0.95) of D band (~1345 cm−1) and G band (~1590 cm−1), illustrating the high graphitization degree (Supplementary Fig. 9). N2 sorption measurement for
FeSA–N–C manifests its high BET surface area up to 1615 m2 g−1 (Supplementary Fig. 10). The mesoporous pore size distribution extends up to 10 nm (Supplementary Fig. 10), which can be also
seen in enlarged TEM image (Fig. 2e). The large surface area and hierarchical pore of FeSA–N–C would make the single Fe atoms readily accessible and guarantee high-flux mass transfer, which
are essential to boost the catalysis42. The existing states of Fe and N elements have been examined by XPS. The N 1 s XPS spectrum of FeSA–N–C is fitted into five configurations, including
pyridinic N (398.5 eV), Fe–N (399.2 eV), pyrrolic N (400.2 eV), graphitic N (401.1 eV), and oxidized N (402.9 eV), respectively (Supplementary Fig. 11a)27,28,29,34,35,36,37,38,43. The Fe 2
p3/2 binding energy in FeSA–N–C centers at 710.5 eV (close to Fe3+), suggesting the positively charged Fe atoms (Supplementary Fig. 11b). No Fe0 belonging to Fe particle can be identified
from XPS spectrum, in consistent with the absence of Fe particles from PXRD and TEM results (Fig. 2d–f, Supplementary Fig. 8). In addition, no obvious Si residual can be detected from the
XPS result of FeSA–N–C (Supplementary Fig. 12). It is noteworthy that the direct pyrolysis of PCN-222(Fe) without SiO2 leads to the formation of Fe nanoparticles in the resultant catalyst
(denoted FeNP–N–C), manifesting the important role of SiO2 in inhibiting the agglomeration of Fe atoms under pyrolysis (Supplementary Fig. 13). The absence of Fe peaks in the XRD pattern of
FeNP–N–C should be due to the small amount of Fe NPs (Supplementary Fig. 8). X-RAY ABSORPTION SPECTROSCOPY STUDIES To gain more information of the electronic structure and coordination
environment of single Fe atoms in FeSA–N–C, X-ray absorption near-edge structure (XANES) and Fourier transform-extended X-ray absorption fine structure (FT-EXAFS) spectra have been examined.
The XANES spectra of Fe in SiO2/FeSA–N–C and FeSA–N–C show almost the same absorption edge located at between Fe foil and Fe2O3 (Fig. 3a), illustrating the positive valence state of Fe
close to +3. The Fourier transformed EXAFS spectra of both SiO2/FeSA–N–C and FeSA–N–C present a dominated peak at ~1.4 Å respecting to the Fe–N scattering path, and no Fe–Fe bond is detected
(Fig. 3b). Furthermore, the curve fitting for EXAFS data of FeSA–N–C further verifies the coordination structure around Fe centers. The best fitting result for the first shell indicates
that Fe atoms are fourfold coordinated by N atoms in average (Fig. 3c, Supplementary Fig. 14, Supplementary Table 4). INSIGHT INTO THE FORMATION PROCESS OF FESA–N–C To unveil the critical
role of the SiO2 nanocasting for the generation of atomically dispersed Fe in FeSA–N–C, as a control, pure PCN-222(Fe) without SiO2 was directly pyrolyzed at 800 °C in N2 atmosphere. The
Fe-based particles with clear Fe–Fe bond are observed in the EXAFS spectrum of FeNP–N–C, in stark contrast to that of FeSA–N–C derived from SiO2@PCN-222(Fe) (Fig. 3b). The results above
unambiguously demonstrate the crucial role of SiO2 in the prevention of Fe atom migration/growth at a high loading under pyrolysis. To further illustrate the stabilizing effect of SiO2 for
Fe atoms in SiO2/FeSA–N–C, the energy changes along the intrinsic reaction coordinate were also investigated through density functional theory (DFT) calculation. By selecting the FeN4 and
FeN4/SiO2 as representative models for FeSA–N–C and SiO2/FeSA–N–C, respectively, the migration energies of each model from the initial state to the final state have been studied in detail
(Supplementary Figs. 15–17). The migration energy barrier of Fe atom in FeN4/SiO2 is found to be 8.35 eV, obviously larger than 6.26 eV of FeN4 without SiO2, suggesting the more thermally
stable Fe atoms in SiO2/FeSA–N–C due to the stabilization effect of SiO2 for Fe atoms (Fig. 3d). Moreover, SiO2 also serves as a hard template, creating much larger surface area of FeSA–N–C
(1615 m2 g−1) than those of FeNP–N–C (445 m2 g−1) and the N-doped porous carbon (simply as N–C, 612 m2 g−1) derived by the pyrolysis of PCN-222 without Fe centers (Supplementary Fig. 10).
Therefore, the SiO2-assisted MOF pyrolysis strategy is able to control the dispersion of active sites and tailor the microstructure of FeSA–N–C, which would be of great significance for
subsequent catalysis. Based on the results above, the formation process of FeSA–N–C from SiO2@PCN-222(Fe) is readily understood. The periodic array of porphyrin linkers in the MOF guarantees
the Fe isolation. The N atoms in porphyrin linker serve as the first safeguard to stabilize the Fe species. Moreover, the SiO2 in the MOF pores behaves as an oxide support to further offer
anchoring effect, upraising migration energy barrier of Fe atoms, and preventing their migration/growth upon pyrolysis (Fig. 3d). Based on the synergistic interactions endowed by the MOF and
SiO2, the thermal agglomeration of Fe atoms is suppressed, leading to the high Fe loadings in atomically dispersed form in FeSA–N–C. In addition to FeSA–N–C, CoSA–N–C, and NiSA–N–C have
also been successfully fabricated from SiO2-nanocasted PCN-222(Co) and PCN-222(Ni), respectively, further manifesting the reliability and universality of this powerful strategy
(Supplementary Fig. 18). ELECTROCATALYTIC PERFORMANCE FOR ORR AND FUEL CELLS Encouraged by the high-content single-atom Fe sites and pore structure of FeSA–N–C, its ORR performance has been
investigated. To our delight, when firstly tested in 0.1 M KOH solution, FeSA–N–C exhibits the highest half-wave potential (_E_1/2 = 0.90 V) and kinetic current density (_J_k) at 0.85 V
(37.19 mA cm−2) among FeNP–N–C, N–C and the commercial Pt/C. The mass activity of FeSA–N–C is calculated to be 21.36 mA g−1 at 0.9 V, much better than FeNP–N–C and N–C counterparts
(Supplementary Table 5). The ideal 4e− transfer process, as well as the excellent durability and methanol tolerance of FeSA–N–C all manifest the superior performance to Pt/C and most
non-noble metal catalysts ever reported under alkaline conditions (Fig. 4a, Supplementary Figs. 19–22, Supplementary Table 6). Encouraged by the excellent ORR performance of FeSA–N–C in
alkaline media, we further explore its performance under more challenging acidic conditions. When tested in 0.1 M HClO4, the linear sweep voltammetry (LSV) curve of FeSA–N–C shows remarkable
ORR activity with much higher _E_1/2 (0.80 V) than that of FeNP–N–C (0.67 V), and N–C (0.51 V; Fig. 4b, c). Also, FeSA–N–C shows better mass activity 1.12 mA g−1 at 0.9 V than that of
FeNP–N–C and N–C counterparts (Supplementary Table 7). In addition, FeSA–N–C demonstrates superior _J_k at 0.80 V (6.14 mA cm−2) to that of FeNP–N–C (0.30 mA cm−2) and N–C (0.17 mA cm−2),
revealing the more favorable kinetics of FeSA–N–C (Fig. 4c). The superior performance of FeSA–N–C to FeNP–N–C and N–C clearly manifests that single-atom Fe sites are the real origin of the
high activity for ORR. It is worth noting that, although much endeavor has been devoted, very limited non-noble metal catalysts were reported to present excellent ORR performance in acidic
media. The results highlight the particular superiority of FeSA–N–C toward ORR among all reported non-noble-metal catalysts (Supplementary Fig. 23, Supplementary Table 8). Given the superb
activity of FeSA–N–C, the deeper ORR investigations have been further conducted in HClO4. To understand the electron transfer mechanism, LSV curves at different rotating rates of rotating
disk electrode are recorded. The Koutechy-Levich (K-L) plots obtained from the LSV curves present good linearity, manifesting the first-order reaction kinetics for FeSA–N–C with a
potential-independent electron transfer rate (Supplementary Fig. 24a)44,45. The electron transfer number calculated by K-L equation is determined to be 4, in accordance with the result of
the rotating ring disk electrode test (Supplementary Fig. 24a, b). Furthermore, the LSV curves of FeSA–N–C after 20,000 cycles, and the methanol addition present its excellent durability and
methanol tolerance, in stark contrast to the significant decline of Pt/C (Supplementary Figs. 25 and 26). The XPS spectrum of Fe for FeSA–N–C after stability test shows identical peak to
the as-prepared catalyst (Supplementary Fig. 27). From the aberration-corrected HAADF-STEM image, Fe atoms in FeSA–N–C after stability test still maintain atomic dispersion on the porous
carbon (Supplementary Fig. 28). The results above manifest that the single Fe atoms in FeSA–N–C can retain the structure after stability test. In addition, to ascertain the critical role of
single Fe atoms for the excellent ORR, SCN−, with strong affinity to Fe, was employed to act as a probe to poison Fe–N sites46,47. Upon the addition of KSCN solution into 0.1 M HClO4, the
deactivation of FeSA–N–C for ORR, with the half-wave potential decreased significantly by 52 mV, clearly manifests that single Fe atoms are responsible for the excellent ORR performance of
FeSA–N–C (Fig. 4d). The excellent ORR activity in acid media of FeSA–N–C has been further approved by the PEMFC measurements. The FeSA–N–C produces a remarkable current density of 292 mA
cm−2 at 0.8 V (or 463 mA cm−2 at 0.8 ViR-free), which is among the highest activities of platinum group metals-free cathodes reported in real PEMFCs (Fig. 4e, Supplementary Fig. 29,
Supplementary Table 9). Moreover, the peak power density reaches a considerable value of 0.68 W cm−2, ~54% the power density of the Pt-cathode under the same operating conditions (Fig. 4e).
The fuel cell stability test for FeSA–N–C indicates a stabilized current density ~0.3 A cm−2 after 20 h testing at 0.5 V (Supplementary Fig. 30). Furthermore, SiO2@FeSA–N–C, featuring much
smaller pore size and volume than FeSA–N–C, has also been tested for fuel cell application (Supplementary Fig. 31). It can be seen that SiO2@FeSA–N–C shows much inferior performance to
FeSA–N–C, clearly demonstrating the vital importance of porous structure in FeSA–N–C for the improvement of fuel cell performance (Supplementary Fig. 32). To better understand the
extraordinary ORR activity of FeSA–N–C, DFT calculations have been performed to obtain the free energy diagrams of FeSA–N–C at equilibrium potential based on the four elementary steps of ORR
in acidic media (Fig. 4f, Supplementary Figs. 33–35, Supplementary Tables 10–12). It is clear that FeSA–N–C and FeNP–N–C show different ORR rate-determining steps. For FeSA–N–C, the
formation of OH* is the most sluggish step with the highest uphill free energy change of 0.55 eV. As for FeNP–N–C, the rate-determining step is the last electron transfer step, requiring the
energy change of 1.25 eV. Obviously, the resistance of ORR on FeSA–N–C is much smaller than that of FeNP–N–C, which well explains the outstanding performance of FeSA–N–C. DISCUSSION In
summary, we have rationally developed a synthetic strategy toward high-loading SACs by nanocasting SiO2 into a MOF, PCN-222(Fe). The 3D skeleton of PCN-222(Fe) realizes the spatial isolation
of Fe atoms that are bound by the N atoms in the linker. More importantly, the SiO2 nanocasted in the MOF mesochannels further offers anchoring effect, generating thermally stable FeN4/SiO2
interfaces and further inhibiting Fe agglomeration under pyrolysis. By integrating these dual protections, the SiO2@PCN-222(Fe) composite, as an ideal precursor, affords FeSA–N–C with a Fe
loading (3.46 wt%) higher than almost all reported single-atom Fe in N-doped carbon materials. Moreover, the synthetic approach is readily extendable to other single metal atoms, such as
CoSA and NiSA. Thanks to the high-content FeSA sites, hierarchical pores and high conductivity of carbon matrix, FeSA–N–C possesses excellent ORR performance in both alkaline and acidic
media, far outperforming all other non-noble-metal catalysts and even the Pt/C. Furthermore, FeSA–N–C delivers excellent performance in acidic PEMFC, demonstrating the great potential of
FeSA–N–C for PEMFC applications. We believe this nanocasting strategy might open up a fascinating avenue to the general fabrication of SACs with high loadings for broad applications. METHODS
SYNTHESIS OF PCN-222(FE) In a typical experiment, ZrOCl2 (108.6 mg), FeTCPPCl (32 mg), and CF3COOH (0.45 mL) were dissolved in DMF (10 mL), and ultrasonically dissolved in a 20 mL Pyrex
vial. The mixture was heated in 120 °C oven for 18 h. After cooling down to room temperature, the obtained dark brown products were separated by centrifugation, and washed subsequently with
DMF for thrice and acetone for twice. The as-obtained precipitates were activated in acetone and finally dried at 60 °C under vacuum overnight. SYNTHESIS OF SIO2@PCN-222(FE) Typically,
PCN-222(Fe) (30 mg) was transferred to a two-necked flask and degassed for 12 h at 130 °C. When the system was cooled down to room temperature, TEOS (1200 μL) was injected into the flask,
and the mixture was sonicated under vacuum for 20 min. Then the obtained solution was centrifuged and the solid was heated at 60 °C under vacuum for 20 min to remove the TEOS on the external
surface of the MOF sample. After that, the sample was exposed to 3 M HCl vapor for 9 h at 60 °C to induce the polycondensation of TEOS within the mesopores of the MOF to give
SiO2@PCN-222(Fe) composite. SYNTHESIS OF FESA–N–C Typically, SiO2@PCN-222(Fe) was heated from the room temperature to 800 °C with a heating rate of 5 °C min−1, then maintained at this
temperature for 2 h in N2 atmosphere. The metal oxides were removed by immersing the sample in the HF (20 wt%) solution for 6 h at 80 °C (Caution! HF is highly toxic and should be handled
very carefully). The black sample was collected by centrifuging, washed several times with distilled water and ethanol, and dried at 60 °C under vacuum overnight. SYNTHESIS OF FENP–N–C
Typically, FeNP–N–C was obtained from the direct pyrolysis of pure PCN-222(Fe) following the same procedure as FeSA–N–C. DATA AVAILABILITY The data that support the findings of this study
are available from the corresponding author upon reasonable request. REFERENCES * Yang, X.-F. et al. Single-atom catalysts: a new frontier in heterogeneous catalysis. _Acc. Chem. Res._ 46,
1740–1748 (2013). Article CAS PubMed Google Scholar * Liu, J. Catalysis by supported single metal atoms. _ACS Catal._ 7, 34–59 (2017). Article CAS Google Scholar * Zhu, C., Fu, S.,
Shi, Q., Du, D. & Lin, Y. Single-atom electrocatalysts. _Angew. Chem. Int. Ed._ 56, 13944–13960 (2017). Article CAS Google Scholar * Zhang, H., Liu, G., Shi, L. & Ye, J.
Single‐atom catalysts: emerging multifunctional materials in heterogeneous catalysis. _Adv. Energy Mater._ 8, 1701343 (2018). Article CAS Google Scholar * Liu, L. & Corma, A. Metal
catalysts for heterogeneous catalysis: from single atoms to nanoclusters and nanoparticles. _Chem. Rev._ 118, 4981–5079 (2018). Article CAS PubMed PubMed Central Google Scholar * Xu,
H., Cheng, D., Cao, D. & Zeng, X. C. A universal principle for a rational design of single-atom electrocatalysts. _Nat. Catal._ 1, 339–348 (2018). Article CAS Google Scholar * Zhang,
H. et al. Dynamic traction of lattice-confined platinum atoms into mesoporous carbon matrix for hydrogen evolution reaction. _Sci. Adv._ 4, eaao6657 (2018). Article ADS PubMed PubMed
Central CAS Google Scholar * Gu, J., Hsu, C., Bai, L., Chen, H. & Hu, X. Atomically dispersed Fe3+ sites catalyze efficient CO2 electroreduction to CO. _Science_ 364, 1091–1094
(2019). Article ADS CAS PubMed Google Scholar * Chen, Y. et al. Single-atom catalysts: synthetic strategies and electrochemical applications. _Joule_ 2, 1242–1264 (2018). Article CAS
Google Scholar * Zhang, L. et al. Graphene defects trap atomic Ni species for hydrogen and oxygen evolution reactions. _Chem_ 4, 285–297 (2018). Article CAS Google Scholar * Liu, P. et
al. Photochemical route for synthesizing atomically dispersed palladium catalysts. _Science_ 352, 797–800 (2016). Article ADS CAS PubMed Google Scholar * Hülsey, M. J., Zhang, J. &
Yan, N. Harnessing the wisdom in colloidal chemistry to make stable single‐atom catalysts. _Adv. Mater._ 30, 1802304 (2018). Article CAS Google Scholar * Yin, P. et al. Single cobalt
atoms with precise N‐coordination as superior oxygen reduction reaction catalysts. _Angew. Chem. Int. Ed._ 55, 10800–10805 (2016). Article CAS Google Scholar * Sun, X. et al. Single
cobalt sites in mesoporous N-doped carbon matrix for selective catalytic hydrogenation of nitroarenes. _J. Catal._ 357, 20–28 (2018). Article CAS Google Scholar * Chen, Y.-Z., Zhang, R.,
Jiao, L. & Jiang, H.-L. Metal-organic framework-derived porous materials for catalysis. _Coord. Chem. Rev._ 362, 1–23 (2018). Article CAS Google Scholar * Zhang, J., Chen, G., Müllen,
K. & Feng, X. Carbon‐rich nanomaterials: fascinating hydrogen and oxygen electrocatalysts. _Adv. Mater._ 30, 1800528 (2018). Article CAS Google Scholar * Furukawa, H., Cordova, K.
E., O’Keeffe, M. & Yaghi, O. M. The chemistry and applications of metal-organic frameworks. _Science_ 341, 1230444 (2013). Article PubMed CAS Google Scholar * Zhou, H.-C. &
Kitagawa, S. Metal-organic frameworks (MOFs). _Chem. Soc. Rev._ 43, 5415–5418 (2014). Article CAS PubMed Google Scholar * Ma, T. Y., Dai, S., Jaroniec, M. & Qiao, S. Z. Metal-organic
framework derived hybrid Co3O4-carbon porous nanowire arrays as reversible oxygen evolution electrodes. _J. Am. Chem. Soc._ 136, 13925–13931 (2014). Article CAS PubMed Google Scholar *
Li, B. et al. Emerging multifunctional metal–organic framework materials. _Adv. Mater._ 28, 8819–8860 (2016). Article ADS CAS PubMed Google Scholar * Islamoglu, T. et al. Postsynthetic
tuning of metal-organic frameworks for targeted applications. _Acc. Chem. Res._ 50, 805–813 (2017). Article CAS PubMed Google Scholar * Jiao, L., Wang, Y., Jiang, H.-L. & Xu, Q.
Metal-organic frameworks as platforms for catalytic applications. _Adv. Mater._ 30, 1703663 (2018). Article CAS Google Scholar * Wang, Y.-R. et al. Oriented electron transmission in
polyoxometalate-metalloporphyrin organic framework for highly selective electroreduction of CO2. _Nat. Commun._ 9, 4466 (2018). Article ADS PubMed PubMed Central CAS Google Scholar *
Li, G., Zhao, S., Zhang, Y. & Tang, Z. Metal-organic frameworks encapsulating active nanoparticles as emerging composites for catalysis: recent progress and perspectives. _Adv. Mater._
30, 1800702 (2018). Article CAS Google Scholar * Zhao, X., Wang, Y., Li, D.-S., Bu, X. & Feng, P. Metal–organic frameworks for separation. _Adv. Mater._ 30, 1705189 (2018). Article
CAS Google Scholar * Jiao, L. & Jiang, H.-L. Metal-organic-framework-based single-atom catalysts for energy applications. _Chem_ 5, 786–804 (2019). Article CAS Google Scholar *
Jiao, L. et al. From metal–organic frameworks to single‐atom Fe implanted N‐doped porous carbons: efficient oxygen reduction in both alkaline and acidic media. _Angew. Chem. Int. Ed._ 57,
8525–8529 (2018). Article CAS Google Scholar * Zhang, H. et al. Single atomic iron catalysts for oxygen reduction in acidic media: particle size control and thermal activation. _J. Am.
Chem. Soc._ 139, 14143–14149 (2017). Article CAS PubMed Google Scholar * Ye, Y. et al. Surface functionalization of ZIF-8 with ammonium ferric citrate toward high exposure of Fe-N active
sites for efficient oxygen and carbon dioxide electroreduction. _Nano Energy_ 38, 281–289 (2017). Article CAS Google Scholar * Jiang, R. et al. Edge-site engineering of atomically
dispersed Fe-N4 by selective C-N bond cleavage for enhanced oxygen reduction reaction activities. _J. Am. Chem. Soc._ 140, 11594–11598 (2018). Article CAS PubMed Google Scholar * Wan, X.
et al. Fe-N-C electrocatalyst with dense active sites and efficient mass transport for high-performance proton exchange membrane fuel cells. _Nat. Catal._ 2, 259–268 (2019). Article CAS
Google Scholar * Chen, Y. et al. Isolated single iron atoms anchored on N‐doped porous carbon as an efficient electrocatalyst for the oxygen reduction reaction. _Angew. Chem. Int. Ed._ 56,
6937–6941 (2017). Article CAS Google Scholar * Abdel-Mageed, A. M. et al. Highly active and stable single-atom Cu catalysts supported by a metal–organic framework. _J. Am. Chem. Soc._
141, 5201–5210 (2019). Article CAS PubMed Google Scholar * Zhang, X. et al. Silica‐protected ultrathin Ni3FeN nanocatalyst for the efficient hydrolytic dehydrogenation of NH3BH3. _Adv.
Energy Mater._ 8, 1702780 (2018). Article CAS Google Scholar * Kang, X. et al. Synthesis of supported ultrafine non‐noble subnanometer‐scale metal particles derived from metal-organic
frameworks as highly efficient heterogeneous catalysts. _Angew. Chem. Int. Ed._ 55, 1080–1084 (2016). Article CAS Google Scholar * Wang, Q. et al. Structural evolution of solid Pt
nanoparticles to a hollow PtFe alloy with a Pt‐skin surface via space‐confined pyrolysis and the nanoscale kirkendall effect. _Adv. Mater._ 28, 10673–10678 (2016). Article CAS PubMed
Google Scholar * Malonzo, C. D. et al. Thermal stabilization of metal-organic framework-derived single-site catalytic clusters through nanocasting. _J. Am. Chem. Soc._ 138, 2739–2748
(2016). Article CAS PubMed Google Scholar * Sa, Y. J. et al. A general approach to preferential formation of active Fe-Nx sites in Fe-N/C electrocatalysts for efficient oxygen reduction
reaction. _J. Am. Chem. Soc._ 138, 15046–15056 (2016). Article CAS PubMed Google Scholar * Feng, D. et al. Zirconium‐metalloporphyrin PCN‐222: mesoporous metal-organic frameworks with
ultrahigh stability as biomimetic catalysts. _Angew. Chem. Int. Ed._ 51, 10307–10310 (2012). Article CAS Google Scholar * Morris, W. et al. Synthesis, structure, and metalation of two new
highly porous zirconium metal-organic frameworks. _Inorg. Chem._ 51, 6443–6445 (2012). Article CAS PubMed Google Scholar * Chen, Y., Hoang, T. & Ma, S. Biomimetic catalysis of a
porous iron-based metal-metalloporphyrin framework. _Inorg. Chem._ 51, 12600–12602 (2012). Article CAS PubMed Google Scholar * Liang, H. W., Zhuang, X., Brüller, S., Feng, X. &
Müllen, K. Hierarchically porous carbons with optimized nitrogen doping as highly active electrocatalysts for oxygen reduction. _Nat. Commun._ 5, 4973 (2014). Article ADS CAS PubMed
Google Scholar * Zhang, Z., Sun, J., Wang, F. & Dai, L. Efficient oxygen reduction reaction (ORR) catalysts based on single iron atoms dispersed on a hierarchically structured porous
carbon framework. _Angew. Chem. Int. Ed._ 57, 9038–9043 (2018). Article CAS Google Scholar * Meng, J. et al. General oriented formation of carbon nanotubes from metal–organic frameworks.
_J. Am. Chem. Soc._ 139, 8212–8221 (2017). Article CAS PubMed Google Scholar * Liang, Y. et al. Covalent hybrid of spinel manganese-cobalt oxide and graphene as advanced oxygen reduction
electrocatalysts. _J. Am. Chem. Soc._ 134, 3517–3523 (2012). Article CAS PubMed Google Scholar * Jiang, W.-J. et al. Understanding the high activity of Fe-N-C electrocatalysts in oxygen
reduction: Fe/Fe3C nanoparticles boost the activity of Fe-Nx. _J. Am. Chem. Soc._ 138, 3570–3578 (2016). Article CAS PubMed Google Scholar * Wang, Q. et al. Phenylenediamine-based
FeNx/C catalyst with high activity for oxygen reduction in acid medium and its active-site probing. _J. Am. Chem. Soc._ 136, 10882–10885 (2014). Article CAS PubMed Google Scholar
Download references ACKNOWLEDGEMENTS L.J., R.Z., and G.W. contributed equally to this work. This work is supported by the NSFC (21725101, 21871244, 21673213, and 21521001), China
Postdoctoral Science Foundation (2019TQ0298 and 2019M660151), International Partnership Program of CAS (211134KYSB20190109), Fundamental Research Funds for the Central Universities
(WK2060030029), BSRF, SSRF, National Synchrotron Radiation Laboratory Foundation (KY2060000160) and Fujian Institute of Innovation (CAS). Use of the Advanced Photon Source is supported by
the U.S. Department of Energy, Office of Science, Office of Basic Energy Sciences, under Contract No. DE-AC02-06CH11357, and the Canadian Light Source and its funding partners. The
calculations in this work are supported by the supercomputing system in the Supercomputing Center of University of Science and Technology of China. AUTHOR INFORMATION Author notes * These
authors contributed equally: Long Jiao, Rui Zhang, Gang Wan. AUTHORS AND AFFILIATIONS * Hefei National Laboratory for Physical Sciences at the Microscale, CAS Key Laboratory of Soft Matter
Chemistry, Collaborative Innovation Center of Suzhou Nano Science and Technology, Department of Chemistry, University of Science and Technology of China, Hefei, Anhui, 230026, People’s
Republic of China Long Jiao, Rui Zhang, Shu-Hong Yu & Hai-Long Jiang * Materials Science Division, Argonne National Laboratory, Lemont, IL, 60439, USA Gang Wan * School of Energy and
Power Engineering, North China Electric Power University, Baoding, 071003, People’s Republic of China Weijie Yang * School of Materials Science and Engineering, Beihang University, Beijing,
100083, People’s Republic of China Xin Wan & Jianglan Shui * X-ray Science Division, Advanced Photon Source, Argonne National Laboratory, Lemont, IL, 60439, USA Hua Zhou Authors * Long
Jiao View author publications You can also search for this author inPubMed Google Scholar * Rui Zhang View author publications You can also search for this author inPubMed Google Scholar *
Gang Wan View author publications You can also search for this author inPubMed Google Scholar * Weijie Yang View author publications You can also search for this author inPubMed Google
Scholar * Xin Wan View author publications You can also search for this author inPubMed Google Scholar * Hua Zhou View author publications You can also search for this author inPubMed Google
Scholar * Jianglan Shui View author publications You can also search for this author inPubMed Google Scholar * Shu-Hong Yu View author publications You can also search for this author
inPubMed Google Scholar * Hai-Long Jiang View author publications You can also search for this author inPubMed Google Scholar CONTRIBUTIONS H.-L.J. conceived the idea and supervised the
project. L.J. and R.Z. performed the experiments, characterizations, and electrochemical measurements. G.W. and H.Z. performed the X-ray absorption spectroscopy measurements and analyses.
W.Y. performed the theoretical calculations. X.W. and J.S. performed the fuel cell measurements. H.-L.J. and L.J. analyzed the data and co-wrote the paper. H.Z., J.S., and S.-H.Y. discussed
the results and commented the manuscript. CORRESPONDING AUTHOR Correspondence to Hai-Long Jiang. ETHICS DECLARATIONS COMPETING INTERESTS The authors declare no competing interests.
ADDITIONAL INFORMATION PEER REVIEW INFORMATION _Nature Communications_ thanks the anonymous reviewer(s) for their contribution to the peer review of this work. PUBLISHER’S NOTE Springer
Nature remains neutral with regard to jurisdictional claims in published maps and institutional affiliations. SUPPLEMENTARY INFORMATION SUPPLEMENTARY INFORMATION RIGHTS AND PERMISSIONS OPEN
ACCESS This article is licensed under a Creative Commons Attribution 4.0 International License, which permits use, sharing, adaptation, distribution and reproduction in any medium or format,
as long as you give appropriate credit to the original author(s) and the source, provide a link to the Creative Commons license, and indicate if changes were made. The images or other third
party material in this article are included in the article’s Creative Commons license, unless indicated otherwise in a credit line to the material. If material is not included in the
article’s Creative Commons license and your intended use is not permitted by statutory regulation or exceeds the permitted use, you will need to obtain permission directly from the copyright
holder. To view a copy of this license, visit http://creativecommons.org/licenses/by/4.0/. Reprints and permissions ABOUT THIS ARTICLE CITE THIS ARTICLE Jiao, L., Zhang, R., Wan, G. _et
al._ Nanocasting SiO2 into metal–organic frameworks imparts dual protection to high-loading Fe single-atom electrocatalysts. _Nat Commun_ 11, 2831 (2020).
https://doi.org/10.1038/s41467-020-16715-6 Download citation * Received: 07 August 2019 * Accepted: 13 May 2020 * Published: 05 June 2020 * DOI: https://doi.org/10.1038/s41467-020-16715-6
SHARE THIS ARTICLE Anyone you share the following link with will be able to read this content: Get shareable link Sorry, a shareable link is not currently available for this article. Copy to
clipboard Provided by the Springer Nature SharedIt content-sharing initiative