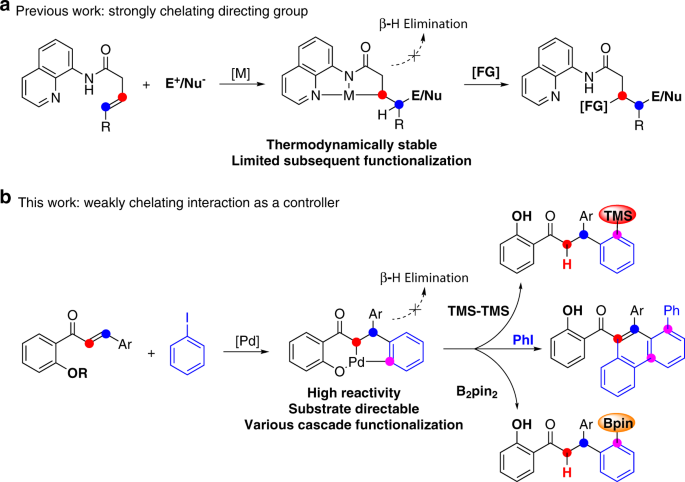
Quadruple c-h activation coupled to hydrofunctionalization and c-h silylation/borylation enabled by weakly coordinated palladium catalyst
- Select a language for the TTS:
- UK English Female
- UK English Male
- US English Female
- US English Male
- Australian Female
- Australian Male
- Language selected: (auto detect) - EN
Play all audios:

ABSTRACT Unlike the well-reported 1,2-difunctionalization of alkenes that is directed by classic pyridine and imine-containing directing groups, oxo-palladacycle intermediates featuring weak
Pd-O coordination have been less demonstrated in C-H activated cascade transformations. Here we report a quadruple C-H activation cascade as well as hydro-functionalization, C-H
silylation/borylation sequence based on weakly coordinated palladium catalyst. The hydroxyl group modulates the intrinsic direction of the Heck reaction, and then acts as an interrupter that
biases the reaction away from the classic β-H elimination and toward C-H functionalization. Mechanistically, density functional theory calculation provides important insights into the key
six-membered oxo-palladacycle intermediates, and indicates that the β-H elimination is unfavorable both thermodynamically and kinetically. In this article, we focus on the versatility of
this approach, which is a strategic expansion of the Heck reaction. SIMILAR CONTENT BEING VIEWED BY OTHERS HYDROGEN-BOND-ACCEPTOR LIGANDS ENABLE DISTAL C(_SP_3)–H ARYLATION OF FREE ALCOHOLS
Article 06 September 2023 PALLADIUM CATALYZED _ORTHO_-C(SP2)–H ACTIVATION/CYCLIZATION OF ARYL AMINES ASSISTED BY IMINE AND VINYLACETIC ACID Article Open access 14 November 2024 MECHANISTIC
STUDY ON THE SIDE ARM EFFECT IN A PALLADIUM/XU-PHOS-CATALYZED ENANTIOSELECTIVE ALKOXYALKENYLATION OF Γ-HYDROXYALKENES Article Open access 22 November 2023 INTRODUCTION The Heck reaction has
become one of the most versatile synthetic tools because of its remarkable tolerance to a variety of functional groups and substrates1,2,3,4,5. In the past few years, studies about the
classic Heck reactions gradually faded out of the vision of researchers while the derived Heck reaction take the lead6,7,8,9,10,11. Among the latter, further transformations of Heck-type
migratory insertion intermediates have attracted much attention because combining the Heck process with other transformations provides a powerful synthetic strategy toward diverse and
complex compounds. To reach this goal, subsequent β–H elimination should be interrupted; it is a typical side reaction in many cascade and 1,2-difunctionalization transformations, and is
extremely hard to avoid because of favorable kinetics12. A typical solution for avoiding β–H elimination is to introduce a blocking group at the β-carbon position13,14,15,16. Other
alternatives include adding a hydrogen source to afford a reductive Heck product17,18,19,20,21,22,23,24, introducing norbornene derivatives in the Catellani reaction25,26,27,28, producing a
π-allyl/benzyl intermediate29,30,31, and oxidizing the Pd(II) to Pd(IV) to create practicable methods32,33,34,35,36,37. Significantly, bidentate nitrogen directing groups have been well
established that prevent β–H elimination in 1,2-difunctionalization and hydro-functionalization reactions via a thermodynamically stable metallacycle38,39,40,41,42,43,44,45,46. However,
thermodynamically stable intermediates engendered by strongly chelating functional groups diminish their reactivity in the subsequent functionalization step47,48. This decreased reactivity
limits the possibilities for cascade transformations, and thus the reactions are limited to mono- and di-functionalization (Fig. 1a). Therefore, we sought a functional group that has
relatively weak coordinating properties that is also present in common substrates. Phenol derivatives are common and typical substrate-directable compounds49,50,51,52. However, their use as
directing groups has only been developed recently53,54,55,56,57, probably because of their weaker σ-donating properties compared with classic imine and pyridine-containing directing
groups47,58,59,60. Apparently, this weaker σ-donation increases the reactivity of the metallacycle intermediate and the difficulty of the first functionalization step. Therefore, we
envisioned a cascade reaction initiated by a Heck-type process, rather than coordination, to avoid low-efficiency functionalization. Subsequently, the judiciously positioned hydroxyl group
stabilized the σ-alkylpalladium intermediate, which is less thermodynamically stable and highly reactive compared to strongly chelating metallacycle complexes, and thereby suppressed β–H
elimination and enabled the multi-component cascade reaction (Fig. 1b). Herein, we report a weaker coordination dominated Pd(0)-catalyzed quadruple C–H activation cascade as well as
hydro-functionalization, C–H silylation, and C–H borylation sequences. By design, the hydroxyl group acts as a controller that dominates the reaction after modulating the intrinsic direction
of the Heck reaction. RESULTS STUDY ON QUADRUPLE C–H ACTIVATION After optimizing the reaction conditions (Supplementary Tables 1 and 2), we examined the substrate scope of quadruple C–H
activation reaction. We first investigated the scope of the alkenes (Fig. 2a). Various 2′-OH-acetophenone substrates (R1) bearing an electron-donating group, a halogen group, or a multiply
substituted group all reacted smoothly to provide the corresponding products in high yields (5–19). Next, we investigated the effect of the substituent R2. As predicted, various alkyl,
halogen, electron-withdrawing, and electron-donating groups were also tolerated. Note that _ortho_-, _meta_-, and multiply substituted R2 were all smoothly converted into final products in
satisfactory yields (20–46). Nevertheless, fused-ring substituents, such as naphthalen-2-yl and phenanthrene-9-yl, were not compatible with our transformation, and afforded an inseparable
complex mixture under the standard reaction conditions. Conversely, heterocyclic substituents showed excellent reactivity, furnishing the desired products in good yields (47–48). A series of
aryl iodide derivatives were subsequently evaluated with this protocol (Fig. 2b). Contrary to our expectations, the reaction was somewhat sensitive to reactants with larger substituents,
such as -4-Et and -4-CO2Me; reactivity was poor and resulted in a complex mixture or no reaction. Gratifyingly, the reaction conditions were sufficiently mild to deliver high yields even
with electron-withdrawing and halogen groups, which provides possibilities for further functionalization (49–53). Aryl bromides also afford the corresponding products, although in much lower
yield. Substrates containing functional groups derived from the hydroxyl group of 1 (R4 = OTIPS, OTBS, OBoc, OAc) were also investigated. Quite interestingly, these derivative substrates
afforded products that were deprotected in situ, in one instance (R4 = OTIPS) in an even higher yield than the model reaction. The molecular structure of products 4, 44, and 50 were verified
by single-crystal X-ray diffraction analyses. STUDY ON C–H SILYLATION The putative six-membered oxo-palladacycle intermediate would be challenging to isolate due to its thermodynamic
instability47. However, we envisaged that this highly reactive species could be captured by other synthetically versatile reagents under our strategy. Encouraged by the extensive
investigations of silylation and borylation in transition-metal-catalyzed C–H activation as well as the subsequent powerful transformations of organosilicon/organoboron compounds61,62,63,64,
we decided to apply our strategy to synthesize a series of silicon- and boron-containing compounds. We first investigated C–H silylation in our cascade reaction with hexamethyldisilane as
the model substrate. Gratifyingly, compound 69 was isolated in 78% yield. Subsequent screening of the reaction conditions with the addition of 1eq of Me4NOAc gave a remarkable increase of
yield (Supplementary Table 3), a result similar to that reported by Zhang65. Notably, this high-efficiency transformation was nearly quantitative at 70 °C. Besides, control experiments
revealed that the added hydrogen mainly came from water (Supplementary Figs. 7–9). We then set out to determine the substrate scope of the C–H silylation protocol. Substitutions of the
alkene moiety were first examined (Fig. 3a). Gratifyingly, common substituents including alkyl, phenyl, halogens, electron-donating groups (55–71), as well as thienyl and ferrocene groups
(72–73) were well-tolerated. The molecular structure of product 73 was verified by X-ray single-crystal diffraction analysis. Next, we extended this silylation from alkenes to aryl iodide
substrates (Fig. 3b). Surprisingly, this cascade process exhibits an extremely efficient transformation and excellent substrate compatibility for various aryl iodide moieties, with nearly
quantitative yields for many of the substrates (74–100). Remarkably, compounds 101 and 102 could be obtained directly from the corresponding aryl iodide derivatives, although in relatively
low yield. STUDY ON C–H BORYLATION Organoboron building blocks are highly versatile, particularly in the organic materials and pharmaceutical fields, with researchers making dramatic
progress on C–H borylation reactions in the past few decades66,67,68. Considering the success with Rh and Ir-catalyzed C–H borylation, and comparatively fewer reports of Pd(0)-catalyzed
cascade reactions69,70,71,72, we subsequently investigated the possibility of C–H borylation under our strategy. After screening a series of reaction conditions, we found that
bis(pinacolato)diboron was an effective boron source. This transformation afforded a satisfactory yield under similar reaction conditions, albeit at a higher temperature, compared with
silylation (Supplementary Table 4). Next, we probed the substrate scope of this C–H borylation process (Fig. 4), various aryl iodides were evaluated first. High yields of the corresponding
products were obtained for _ortho-_ and _para-_substituted aryl iodide derivatives (104–119), especially for substrates containing electron-donating-groups (109, 118, 119). Conversely,
substituted aryl iodides containing strongly electron-withdrawing groups (such as trifluoromethyl-, nitro-, and polyhalogen-) were not compatible, which resulted in inseparable complex
mixtures or no reaction. We then investigated several representative alkenes using 2-iodotoluene as a representative aryl iodide. Although the average yields of these compounds were
comparatively lower (120–128), the heterocyclic aldehyde substrates exhibited a satisfactory result (129–131). The exact structures of products 103, 105, and 131 were verified by X-ray
single-crystal diffraction analysis. Overall, these transformations exhibit extremely high selectivity and excellent compatibility with a wide variety of functional groups. No simple β–H
elimination products were detected for any of the examples in Figs. 2–4, and it thus represents a powerful strategic expansion of the Heck reaction. FURTHER STUDY The potential synthetic
applications of these cascade reactions were subsequently investigated, starting with a gram-scale experiment for the quadruple C–H activation cascade. The yield was slightly decreased at
the 5- and 10-mmol scale under the standard conditions, affording product 4 in 72 and 60% yields, respectively. The hydroxyl group was easily further transformed into other functional
groups. Compounds 136 and 137 were efficiently obtained in 90 and 85% yields, respectively (Fig. 5a, b). Interestingly, the fluoro-substituted product presented excellent reactivity with the
carbazole derivatives and gave an almost quantitative yield of 138, 139, and 140 (Fig. 5c). Moreover, the emission spectra of 138 and 139 presented a notable solvatochromic effect. A
distinct red shift was observed, with increasing solvent polarity (430 nm in n-hexane to 520 nm in DMSO for 138; 430 nm in n-hexane to 505 nm in DMSO for 139), which is a typical CT (charge
transfer) characteristic in the excited state (Fig. 6)73,74. Further studies toward CT and possible ESIPT (excited state intramolecular proton transfer) properties of these compounds are
currently underway. MECHANISTIC INVESTIGATION To gain insight into the mechanism of this domino reaction, a series of control experiments were conducted (Fig. 7). First, we investigated
whether the hydroxyl group was necessary under standard conditions. No reaction occurred when we replaced the −OH with −H, −OMe, −OBu, −OBn, or −OMEM, which indicated that the hydroxyl group
is necessary. Similarly, an inseparable complex mixture was obtained when the -OH was replaced with −NH2 or −NHAc, which suggests that this transformation is highly specific to the hydroxyl
group. In addition, we investigated the influence position of the HO-group on the aromatic ring on the reaction. As expected, only the _ortho_-substituted substrate afforded a smooth
reaction whereas the _meta_- and _para_-substituted substrates gave Heck products in addition to unreacted starting materials, which strongly suggests an intramolecular coordination between
the hydroxyl group and the metal center. As previously discussed, replacing –OH with groups such as −OTIPS, −OTBS, and −OBoc gave deprotected final products, indicative of a probable
six-membered oxo-palladacycle intermediate and an X-type coordination mode (Fig. 7a)55,75. To further investigate the potential coordination modes of the hydroxyl group with the palladium
center, the cesium salt of 1 was investigated as substrate under the standard reaction conditions. Compound 4 was isolated in 86% yield (Fig. 7b), which suggested that the hydroxyl group
serves as an anionic ligand. Interestingly, when we added 5 equiv. of n-BuCl to the reaction, we did not detect product, and instead, compound 141 was isolated in 13% yield along with 62%
nucleophilic attack product. The exact structure of 141 was verified by X-ray single-crystal diffraction analysis. This control experiment revealed that dehydrogenation is probably the last
step of the entire transformation. Compound 141 probably resulted from a long-lived intermediate that is captured by n-BuCl before dehydrogenation (Fig. 7c). Submission of 141 to the
standard reaction conditions delivered a complex mixture and significant amounts of recovered starting material. This suggested that the hydroxyl group is important to the dehydrogenation
step (Fig. 7d). To verify our hypothesis, deprotected compound 142 was prepared. Gratifyingly, transformation of 142 under standard reaction conditions (3 h) gave 4 in almost quantitative
yield. However, if the base was left out under otherwise standard conditions, only 28% yield of 4 (12 h) was obtained. The yield of 4 also clearly decreased upon removal of PhI from the
standard reaction conditions. Only trace amounts of 4 could be detected upon removing the palladium catalyst, irrespective of whether an Ar or O2 atmosphere was used (Fig. 7e). In summary,
the hydroxyl group, palladium catalyst, and base are essential to the dehydrogenation process. The excess PhI may act as a potential oxidant of the palladium catalyst, as per GC-MS analysis.
The lack of hydrogen gas evolution further supports this mechanism (Supplementary Figs. 1 and 2). Next, when 143 was used as substrate, no desired product was obtained (Fig. 7f), which
indicates that the electronics of double bond, as well as the potential intramolecular hydrogen bonding interactions between the hydroxyl and carbonyl groups may be important in the
reaction. In addition, the possibility of coordination between palladium center and carbonyl group should be taken into consideration. Finally, a series of isotopic labeling experiments were
examined and the outcomes indicated that the hydrogen atom of the reconstituted hydroxyl group of the final product is derived from various moieties (Supplementary Figs. 3–6). An
intramolecular hydrogen atom transfer process was not involved in this transformation (for selected control experiments of C–H silylation, see Supplementary Fig. 10). THEORETICAL CALCULATION
STUDY Based on the previous studies about the Saegusa-Ito oxidation76,77,78,79 and our experimental findings, we performed density functional theory calculations to further complement the
mechanism of the final dehydrogenation (Fig. 8). According to the calculations, the PdII species that is oxidized by excess PhI coordinates with 142 to form complex IV, followed by ligand
metathesis to give complex V via a four-membered cyclic transition state with a 25.3 kcal mol−1 free energy barrier (IV-V). In light of the essential nature of the carbonyl group during the
reaction (Fig. 7f), we proposed that the possibility of coordination between palladium center and carbonyl group should not be overlooked in the process of dehydrogenation. Under this
circumstance, after a ligand exchange from complex V, two different hydrogen abstraction pathways are taken into consideration. First, the palladium center may coordinate with two acetates
that act as monodentate ligand, then α-H abstraction may occur by the proximal acetate via TS′VI-VII (Path B), along with the extrusion of one HOAc, followed by isomerization to afford
complex VII. The activation energy of this pathway is calculated to be 32.9 kcal mol−1 with respect to VI (VI-VII). Second, the possibility of hydrogen abstraction by free acetate ion could
not be excluded. In this case, α-H abstraction of complex VI may occur via TSVI−VII by the free acetate ion (Path A), followed by isomerization to form complex VII. Comparatively, this
pathway requires an activation energy of 20.5 kcal mol−1 with respect to VI (VI-VII), and 12.4 kcal mol−1 lower than that of the former pathway. Upon the tautomerism of VII from enol to
keto-form, a more stable complex VIII (15.8 kcal mol−1 more stable than VII) is formed by ligand exchange. Then a _syn_-β-H elimination affords a Pd(II)-hydride complex IX. Interestingly,
the direct O−H reductive elimination need to bear a free energy barrier of 28.3 kcal mol−1. Alternatively, anionic complex IX can form a hydrogen bond with acetic acid to form complex X
reversibly. Sequentially, a proton transfer takes place via transition state TSX−II. The calculated free energy barrier for this step is only 15.2 kcal mol−1. After ligand exchange, product
4 could be yielded with the regeneration of catalytically active complex II. To further understand the formation of the key six-membered oxo-palladacycle intermediate in catalytic cycle, a
corresponding computational study was conducted (Fig. 9). The formation of complex V′ involves oxidative addition and _syn_-migratory insertion steps. The higher thermodynamic stability of
complex V′ may be partially attributed to the coordinated interaction between hydroxyl and palladium center. The process from 1 to V′ is both feasible (ΔG≠max = 18.6 kcal mol−1) and
favorable (ΔG = −1.7 kcal mol−1). In terms of substrate scope, no simple β–H elimination products were detected for any of the examples in Figs. 2–4, presenting an efficient interruption of
the classic Heck reaction pathway. Therefore, we hoped to elucidate the origin of this selectivity by calculating both pathways. According to the calculations, the β–H elimination occurs via
TSV′−VII′B and requires an activation energy of 9.8 kcal mol−1 with respect to complex V′, and the corresponding product VIII′B is formed with an endergonicity of 3.3 kcal mol−1 with
respect to complex V′. Comparatively, the energy barrier for the formation of six-membered oxo-palladacycle via TSVI′−VII′A is only 6.6 kcal mol−1 with respect to complex V′, 3.2 kcal mol−1
lower than that of the competing β–H elimination pathway. Besides, the corresponding complex VII′A is formed with an exergonicity of 14.3 kcal mol−1 with respect to complex V′. More
importantly, complex VII′A is 17.6 kcal mol−1 more stable than VIII′B. Therefore, the β–H elimination pathway is unfavorable from both thermodynamic and kinetic viewpoints. Moreover, when
complex V′ is formed, one phenyl group coming from iodobenzene coordinates onto Pd leading to the discrimination of another one. After a ligand exchange with acetate, a more stable complex
VI′ can be formed, where the coordination of phenyl group is remained. We found that the dissociation of phenyl group by ligand exchange with another oxygen atom in coordinated acetate need
to bear a free energy barrier of 19.1 kcal mol−1 via transition state TSVI′−VII′C. As a contrast, the activation free energy for the alternative proton abstraction via a six-membered
transition state (TSVI′−VII′A) to afford complex VII′A is only 15.4 kcal mol−1. Therefore, the exchange of two phenyl groups cannot be achieved. Next, the extrusion of HOAc affords the
highly reactive complex VIII′A, followed by a CMD process (TSVIII′A−IX′) to deliver the key intermediate IX′. Note that this C–H activation step is highly feasible, with the energy barrier
was calculated to be 13.7 kcal mol−1 (for computational details, see Supplementary Data 1). DISCUSSION In conclusion, we have developed a weaker coordination dominated Pd(0)-catalyzed
quadruple C–H activation cascade as well as hydro-functionalization, C–H silylation, and C–H borylation sequences with broad substrate scope, good yields, and excellent chemo-selectivity.
Relatively weak coordination between the hydroxyl group and metal is the key factor for these cascade transformations. DFT calculations indicate that the β–H elimination is unfavorable based
on thermodynamic and kinetic considerations and also rationalize the final dehydrogenation process of the quadruple C–H activation cascade. This strategy represents a generally applicable
approach that is compatible with other functionalization processes such as C–H silylation and borylation, which represent a strategic expansion of the Heck reaction. Further studies toward
additional potentially useful properties of these compounds are in progress. METHODS GENERAL PROCEDURE FOR SYNTHESIS OF 4 A 25 ml Schlenk-type tube was charged with the mixture of alkene
(0.1 mmol), iodobenzene (0.4 mmol), Pd(OAc)2 (5 mol%), Na2CO3 (0.2 mmol), H2O (40 μL) in DMF (2 mL). The reaction was frozen with the liquid nitrogen and then the tube was evacuated and
backfilled with nitrogen for five times. The mixture was first stirred at room temperature for 10 min and then stirred at 110 °C for 12 h. After cooling to room temperature, the mixture was
quenched with water, extracted with EtOAc, and concentrated under reduced pressure. The crude product was purified by column chromatography on silica gel (eluent: petroleum ether/EtOAc =
100/1) to afford the products 4. DATA AVAILABILITY The authors declare that all the data supporting the findings of this study are available within the article and Supplementary Information
files, and are also available from the corresponding author upon reasonable request. The X-ray crystallographic coordinate for structure reported in this study have been deposited at the
Cambridge Crystallographic Data Centre (CCDC) under deposition numbers 1970136 (3), 1964456 (4), 1961529 (44), 1961532 (50), 1969923 (73), 1969916 (82), 1969930 (103), 1969931 (105), 1974984
(131) and 1961844 (141). These data could be obtained free of charge from The Cambridge Crystallographic Data Center via http://www.ccdc.cam.ac.uk/data_request/cif. REFERENCES * Heck, R.-F.
Palladium-catalyzed reactions of organic halides with olefins. _Acc. Chem. Res._ 12, 146–151 (1979). Article CAS Google Scholar * Fahey, D.-R. & Mahan, J.-E. Reversible oxidative
addition of triphenylphosphine to zero-valent nickel and palladium complexes. _J. Am. Chem. Soc._ 98, 4499–4503 (1976). Article CAS Google Scholar * Stille, J.-K. & Lau, K.-S.
Oxidative addition of alkyl halides to zero-valent palladium complexes. mechanisms. _J. Am. Chem. Soc._ 98, 5841–5849 (1976). Article CAS Google Scholar * Milstein, D. & Stille, J.-K.
Mechanism of reductive elimination. Reaction of alkylpalladium (II) complexes with tetraorganotin, organolithium, and Grignard reagents. evidence for palladium (IV) intermediacy. _J. Am.
Chem. Soc._ 101, 4981–4991 (1979). Article CAS Google Scholar * Hegedus, L.-S. & Williams, R.-E. Palladium-assisted alkylation of olefins. _J. Am. Chem. Soc._ 102, 4973–4979 (1980).
Article CAS Google Scholar * Beletskaya, I.-P. & Cheprakov, A.-V. The Heck reaction as a sharpening stone of palladium catalysis. _Chem. Rev._ 100, 3009–3066 (2000). Article CAS
Google Scholar * Delcamp, J.-H., Brucks, A.-P. & White, M.-C. A general and highly selective chelate-controlled intermolecular oxidative Heck reaction. _J. Am. Chem. Soc._ 130,
11270–11271 (2008). Article CAS Google Scholar * Matsubara, R., Gutierrez, A.-C. & Jamison, T.-F. Nickel-catalyzed Heck-type reactions of benzyl chlorides and simple olefins. _J. Am.
Chem. Soc._ 133, 19020–19023 (2011). Article CAS Google Scholar * Bloome, K.-S., Mcmahen, R.-L. & Alexanian, E.-J. Palladium-catalyzed Heck-type reactions of alkyl iodides. _J. Am.
Chem. Soc._ 133, 20146–20148 (2011). Article CAS Google Scholar * Wu, C.-L. & Zhou, J.-R. Asymmetric intermolecular Heck reaction of aryl halides. _J. Am. Chem. Soc._ 136, 650–652
(2014). CAS Google Scholar * Parasram, M., Iaroshenko, V.-O. & Gevorgyan, V. Endo-selective Pd-catalyzed silyl methyl Heck reaction. _J. Am. Chem. Soc._ 136, 17926–17929 (2014). CAS
Google Scholar * Lan, Y., Liu, P. & Lautens, M. Theoretical study of Pd(0)-catalyzed carbohalogenation of alkenes: mechanism and origins of reactivities and selectivities in alkyl
halide reductive elimination from Pd (II) species. _Chem. Sci._ 3, 1987–1995 (2012). CAS Google Scholar * Piou, T., Neuville, L. & Zhu, J.-P. Activation of a C(sp3)-H bond by a
transient σ-alkylpalladium(II) complex: synthesis of spirooxindoles through a palladium-catalyzed domino carbopalladation/C(sp3)-C(sp3) bond-forming process. _Angew. Chem. Int. Ed._ 51,
11561–11565 (2012). CAS Google Scholar * Petrone, D.-A., Yen, A. & Lautens, M. Dearomative indole bisfunctionalization via a diastereoselective palladium-catalyzed arylcyanation. _Org.
Lett._ 17, 4838–4841 (2015). CAS Google Scholar * Liu, R.-R., Wang, Y.-G. & Jia, Y.-X. Enantioselective dearomative difunctionalization of indoles by palladium-catalyzed
Heck/Sonogashira sequence. _Angew. Chem. Int. Ed._ 56, 7475–7478 (2017). CAS Google Scholar * Xu, X.-B., Liu, J.-C. & Yin, B.-L. Pd-catalyzed regioselective intramolecular direct
arylation of 3-indolecarboxamides: access to spiro-indoline-3,3′-oxindoles and 5,11-dihydro-6H-indolo[3,2-c]quinolin-6-ones. _Chem. Commun._ 53, 7796–7799 (2017). CAS Google Scholar *
Cacchi, S. & Arcadi, A. Palladium-catalyzed conjugate addition type reaction of aryl iodides with α, β-unsaturated ketones. _J. Org. Chem._ 48, 4236–4240 (1983). CAS Google Scholar *
Cacchi, S. & Palmieri, G. The palladium-catalyzed conjugate addition type reaction of aryl iodides with α, β-unsaturated aldehydes. _J. Organomet. Chem._ 268, C48–C51 (1984). CAS Google
Scholar * Hagiwara, H., Eda, Y. & Ito, N. Selectivity in palladium catalyzed arylation: synthetic application leading to aromatized ionone natural products. _Tetrahedron Lett._ 39,
4055–4058 (1998). CAS Google Scholar * Tobrman, T. & Dvorak, D. Reductive heck reaction of 6-halopurines. _Tetrahedron Lett._ 45, 273–276 (2004). CAS Google Scholar * Geoghegan, K.,
Kelleher, S. & Evans, P. An investigation into the one-pot Heck olefination–hydrogenation reaction. _J. Org. Chem._ 76, 2187–2194 (2011). CAS Google Scholar * Gao, P. & Cook, S.-P.
A Reductive-Heck approach to the hydroazulene ring system: a formal synthesis of the englerins. _Org. Lett._ 14, 3340–3343 (2012). CAS Google Scholar * Yang, F.-Y., Jin, Y.-X. & Wang,
C. Nickel-catalyzed asymmetric intramolecular reductive Heck reaction of unactivated alkenes. _Org. Lett._ 21, 6989–6994 (2019). CAS Google Scholar * Oxtoby, L.-J., Gurak, J.-A. &
Engle, K.-M. Palladium-catalyzed reductive Heck coupling of alkenes. _Trends Chem._ 1, 572–587 (2019). Google Scholar * Catellani, M. A complex catalytic cycle leading to a regioselective
synthesis of _o,o_′-disubstituted vinylarenes. _Angew. Chem. Int. Ed._ 36, 119–122 (1997). CAS Google Scholar * Bressy, C., Alberico, D. & Lautens, M. A route to annulated indoles via
a palladium-catalyzed tandem alkylation/direct arylation reaction. _J. Am. Chem. Soc._ 127, 13148–13149 (2005). CAS Google Scholar * Wang, X.-C., Engle, K.-M. & Yu, J.-Q.
Ligand-enabled _meta_-C-H activation using a transient mediator. _Nature_ 519, 334–338 (2015). ADS CAS Google Scholar * Gao, Q.-W., Shang, Y. & Zhou, Q.-H. Modular dual-tasked C-H
methylation via the catellani strategy. _J. Am. Chem. Soc._ 141, 15986–15993 (2019). CAS Google Scholar * Yamamoto, E., Toste, F.-D. & Sigman, M.-S. Development and analysis of a
Pd(0)-catalyzed enantioselective 1,1-diarylation of acrylates enabled by chiral anion phase transfer. _J. Am. Chem. Soc._ 138, 15877–15880 (2016). CAS Google Scholar * Liu, J.-C., Jiang,
H.-F. & Yin, B.-L. Diastereospecific and enantioselective access to dispirooxindoles from furfurylcyclobutanols by means of a Pd-catalyzed arylative dearomatization/ring expansion
cascade. _Org. Lett._ 18, 6440–6443 (2016). Article CAS Google Scholar * Liu, J.-C., Jiang, H.-F. & Yin, B.-L. Palladium-catalyzed dearomatizing 2,5-alkoxyarylation of furan rings:
diastereospecific access to spirooxindoles. _Chem. Commun._ 52, 9550–9553 (2016). Article CAS Google Scholar * Xu, L.-M., Li, B.-J. & Shi, Z.-J. Organopalladium (IV) chemistry. _Chem.
Soc. Rev._ 39, 712–733 (2010). Article CAS Google Scholar * Muniz, K. High-oxidation-state palladium catalysis: new reactivity for organic synthesis. _Angew. Chem. Int. Ed._ 48,
9412–9423 (2009). Article CAS Google Scholar * Hickman, A.-J. & Sanford, M.-S. High-valent organometallic copper and palladium in catalysis. _Nature_ 484, 177–185 (2012). Article ADS
CAS Google Scholar * Yin, G.-L., Mu, X. & Liu, G.-S. Palladium(II)-catalyzed oxidative difunctionalization of alkenes: bond forming at a high-valent palladium center. _Acc. Chem.
Res._ 49, 2413–2423 (2016). Article CAS Google Scholar * Park, H., Hong, Kai & Controlling, Yu,J.-Q. Pd(IV) reductive elimination pathways enables Pd(II)-catalysed enantioselective
C(sp3)-H fluorination. _Nat. Chem._ 10, 755–762 (2018). Article CAS Google Scholar * Mauleon, P. & Carretero, J.-C. Palladium-catalyzed cascade reaction of α, β-unsaturated sulfones
with aryl iodides. _Chem. Eur. J._ 9, 1511–1520 (2003). Article CAS Google Scholar * He, G. & Chen, G. A practical strategy for the structural diversification of aliphatic scaffolds
through the palladium-catalyzed picolinamide-directed remote functionalization of unactivated C(sp3)-H bonds. _Angew. Chem. Int. Ed._ 50, 5192–5196 (2011). Article CAS Google Scholar *
Ano, Y., Tobisu, M. & Chatani, N. Palladium-catalyzed direct ethynylation of C(sp3)-H bonds in aliphatic carboxylic acid derivatives. _J. Am. Chem. Soc._ 133, 12984–12986 (2011). Article
CAS Google Scholar * He, G., Zhao, Y.-S. & Chen, G. Highly efficient syntheses of azetidines, pyrrolidines, and indolines via palladium catalyzed intramolecular amination of C(sp3)-H
and C(sp2)-H Bonds at γ and δ positions. _J. Am. Chem. Soc._ 134, 3–6 (2012). CAS Google Scholar * Rouquet, G. & Chatani, N. Catalytic functionalization of C(sp2)-H and C(sp3)-H bonds
by using bidentate directing groups. _Angew. Chem. Int. Ed._ 52, 2–20 (2013). Google Scholar * O’Duill, M.-L., Liu, P. & Engle, K.-M. Tridentate directing groups stabilize 6-membered
palladacycles in catalytic alkene hydrofunctionalization. _J. Am. Chem. Soc._ 139, 15576–15579 (2017). Google Scholar * Engle, K.-M. Nickel-catalyzed β,γ-dicarbofunctionalization of alkenyl
carbonyl compounds via conjunctive cross-coupling. _J. Am. Chem. Soc._ 139, 10657–10660 (2017). Google Scholar * Wang, C.-D., Xiao, G.-L. & Loh, T.-P. Palladium-catalyzed
regiocontrollable reductive Heck reaction of unactivated aliphatic alkenes. _J. Am. Chem. Soc._ 140, 9332–9336 (2018). CAS Google Scholar * Wang, H., Peng, Q. & Chen, G.
Palladium-catalyzed amide-directed enantioselective hydrocarbofunctionalization of unactivated alkenes using a chiral monodentate oxazoline ligand. _J. Am. Chem. Soc._ 140, 3542–3546 (2018).
CAS Google Scholar * Liu, Z., Derosa, J. & Engle, K.-M. Palladium (II)-catalyzed regioselective syn-hydroarylation of disubstituted alkynes using a removable directing group. _J. Am.
Chem. Soc._ 138, 13076–13081 (2016). CAS Google Scholar * Engle, K.-M., Mei, T.-S. & Yu, J.-Q. Weak coordination as a powerful means for developing broadly useful C-H functionalization
reactions. _Acc. Chem. Res._ 45, 788–802 (2012). CAS Google Scholar * Li, G., Wan, L. & Yu, J.-Q. Pd(II)-catalyzed C-H functionalizations directed by distal weakly coordinating
functional groups. _J. Am. Chem. Soc._ 137, 4391–4397 (2015). CAS Google Scholar * Suter, C.-M. Relationships between the structures and bactericidal properties of phenols. _Chem. Rev._
28, 269–299 (1941). Article CAS Google Scholar * Fu, X., Schmitz, F.-J. & Schatzman, R.-C. Enzyme inhibitors: new and known polybrominated phenols and diphenyl ethers from four
indo-pacific dysidea sponges. _J. Nat. Prod._ 58, 1384–1391 (1995). CAS Google Scholar * Koganov, M., Dueva, O. & Tsorin, B.-L. Activities of plant-derived phenols in a fibroblast cell
culture model. _J. Nat. Prod._ 62, 481–483 (1999). CAS Google Scholar * Davis, R.-A., Hofmann, A. & Poulsen, S.-A. Natural product-based phenols as novel probes for mycobacterial and
fungal carbonic anhydrases. _J. Med. Chem._ 54, 1682–1692 (2011). CAS Google Scholar * Satoh, T., Miura, M. & Nomura, M. Palladium-catalyzed regioselective mono- and diarylation
reactions of 2-phenylphenols and naphthols with aryl halides. _Angew. Chem. Int. Ed._ 36, 1740–1742 (1997). CAS Google Scholar * Terao, Y., Miura, M. & Nomura, M. Palladium-catalyzed
arylative carbon-carbon bond cleavage of α,α-disubstituted arylmethanols. _J. Am. Chem. Soc._ 123, 10407–10408 (2001). CAS Google Scholar * Xiao, B., Gong, T.-J. & Liu, L. Synthesis of
dibenzofurans via palladium-catalyzed phenol-directed C-H activation/C-O cyclization. _J. Am. Chem. Soc._ 133, 9250–9253 (2011). CAS Google Scholar * Thirunavukkaras, V.-S. &
Ackermann, L. Hydroxyl-directed ruthenium-catalyzed C-H bond functionalization: versatile access to fluorescent pyrans. _Org. Lett._ 14, 3416–3419 (2012). Google Scholar * Zhang, C., Ji, J.
& Sun, P.-P. Palladium-catalyzed alkenylation via sp2 C-H bond activation using phenolic hydroxyl as the directing group. _J. Org. Chem._ 79, 3200–3205 (2014). CAS Google Scholar *
Cope, A.-C. & Siekman, R. Formation of covalent bonds from platinum or palladium to carbon by direct substitution. _J. Am. Chem. Soc._ 87, 3272–3273 (1965). CAS Google Scholar * Cope,
C. & Friedrich, E.-C. Electrophilic aromatic substitution reactions by platinum (II) and palladium (II) chlorides on N,N-dimethylbenzylamines. _J. Am. Chem. Soc._ 90, 909–913 (1968). CAS
Google Scholar * Constable, A.-G., Mcdonald, W.-S. & Shaw, B.-L. Palladation of dimethylhydrazones, oximes, and oxime _O_-allyl ethers: crystal structure of [Pd3(ON = CPriPh)6]. _J.
Chem. Soc. Chem. Commun_. 1978, 1061–1062 (1978). Google Scholar * Zhang, F., Wu, D. & Xu, Y. Thiophene-based conjugated oligomers for organic solar cells. _J. Mater. Chem._ 21,
17590–17600 (2011). CAS Google Scholar * Showell, G.-A. & Mills, J.-S. Chemistry challenges in lead optimization: silicon isosteres in drug discovery. _Drug. Discov. Today_ 8, 551–556
(2003). CAS Google Scholar * Franz, A.-K. & Wilson, S.-O. Organosilicon molecules with medicinal applications. _J. Med. Chem._ 56, 388–405 (2013). CAS Google Scholar * Langkopf, E.
& Schinzer, D. Uses of silicon-containing compounds in the synthesis of natural products. _Chem. Rev._ 95, 1375–1408 (1995). CAS Google Scholar * Zhou, B., Shao, C.-D. & Zhang,
Y.-H. Palladium-catalyzed sequential three-component reactions to access vinylsilanes. _Chem. Commun._ 54, 10598–10601 (2018). CAS Google Scholar * Yang, C.-T., Marder, T.-B. & Liu, L.
Alkylboronic esters from copper-catalyzed borylation of primary and secondary alkyl halides and pseudohalides. _Angew. Chem. Int. Ed._ 51, 528–532 (2012). CAS Google Scholar * Legare,
M.-A. & Fontaine, F.-G. Metal-free catalytic C-H bond activation and borylation of heteroarenes. _Science_ 349, 513–516 (2015). ADS CAS Google Scholar * Lv, J.-H., Houk, K.-N. &
Shi, Z.-Z. Metal-free directed sp2-C-H borylation. _Nature_ 575, 336–340 (2019). ADS CAS Google Scholar * Mkhalid, I.-A., Coventry, D.-N. & Marder, T.-B. Ir-catalyzed borylation of
C-H bonds in N-containing heterocycles: regioselectivity in the synthesis of heteroaryl boronate esters. _Angew. Chem. Int. Ed._ 45, 489–491 (2006). CAS Google Scholar * Ohmura, T.,
Kijima, A. & Suginome, M. Synthesis of 1-borylisoindoles via palladium-catalyzed dehydrogenation/C-H borylation of isoindolines. _J. Am. Chem. Soc._ 131, 6070–6071 (2009). Article CAS
Google Scholar * Dai, H.-X. & Yu, J.-Q. Pd-catalyzed oxidative _ortho-_C-H borylation of arenes. _J. Am. Chem. Soc._ 134, 134–137 (2012). Article CAS Google Scholar * Hu, T.-J.,
Feng, C.-G. & Lin, G.-Q. Borylation of olefin C-H bond via aryl to vinyl palladium 1,4-migration. _J. Am. Chem. Soc._ 138, 2897–2900 (2016). Article CAS Google Scholar * Li, B.-J.,
Zhou, L. & You, J.-S. Unexpected sole enol-form emission of 2-(2′-hydroxyphenyl)oxazoles for highly efficient deep-blue-emitting organic electroluminescent devices. _Adv. Funct. Mater._
27, 1605245 (2017). Article CAS Google Scholar * Mutai, T., Houjou, H. & Ogura, M. Development of imidazo[1,2-_a_]pyridine derivatives with an intramolecular hydrogen-bonded
seven-membered ring exhibiting bright ESIPT luminescence in the solid state. _Org. Lett._ 21, 2143–2146 (2019). Article CAS Google Scholar * Lu, Y., Wang, D.-H. & Yu, J.-Q. Pd
(II)-catalyzed hydroxyl-directed C-H olefination enabled by monoprotected amino acid ligands. _J. Am. Chem. Soc._ 132, 5916–5921 (2010). Article CAS Google Scholar * Ito, Y., Hirao, T.
& Saegusa, T. Synthesis of α,β-unsaturated carbonyl compounds by palladium (II)-catalyzed dehydrosilylation of silyl enol ethers. _J. Org. Chem._ 43, 1011–1013 (1978). Article CAS
Google Scholar * Lu, Y., Nguyen, P.-L. & Lebel, H. Palladium-catalyzed Saegusa-Ito oxidation: synthesis of α,β-unsaturated carbonyl compounds from trimethylsilyl enol ethers. _J. Org.
Chem._ 78, 776–779 (2013). Article CAS Google Scholar * Hirao, T. Synthetic strategy: palladium-catalyzed dehydrogenation of carbonyl compounds. _J. Org. Chem._ 84, 1687–1692 (2019).
Article CAS Google Scholar * Chen, H., Chen, J. & Chen, T. Direct dehydrogenation for the synthesis of α,β-unsaturated carbonyl compounds. _Adv. Synth. Catal._ 362, 3332–3346 (2020).
Article CAS Google Scholar Download references ACKNOWLEDGEMENTS This work was supported by the National Natural Science Foundation of China (Grants 21971080, 21971079, 21602070 and
21772051). This work was supported by ‘The Fundamental Research Funds for the Central Universities’ (CCNU15ZX002 and CCNU18QN011). This work was also supported by Henan Province
Supercomputing Center. AUTHOR INFORMATION AUTHORS AND AFFILIATIONS * Key Laboratory of Pesticide & Chemical Biology, Ministry of Education, College of Chemistry, Central China Normal
University, Wuhan, 430079, PR China Bo-Cheng Tang, Xiang-Long Chen, Cai He, Jin-Tian Ma, Yan-Dong Wu & An-Xin Wu * College of Chemistry and Institute of Green Catalysis, Zhengzhou
University, 100 Science Avenue, Zhengzhou, 450001, Henan, PR China Wen-Xuan Lin & Yu Lan * School of Chemistry and Chemical Engineering, and Chongqing Key Laboratory of Theoretical and
Computational Chemistry, Chongqing University, Chongqing, 400030, PR China Yu Lan Authors * Bo-Cheng Tang View author publications You can also search for this author inPubMed Google Scholar
* Wen-Xuan Lin View author publications You can also search for this author inPubMed Google Scholar * Xiang-Long Chen View author publications You can also search for this author inPubMed
Google Scholar * Cai He View author publications You can also search for this author inPubMed Google Scholar * Jin-Tian Ma View author publications You can also search for this author
inPubMed Google Scholar * Yan-Dong Wu View author publications You can also search for this author inPubMed Google Scholar * Yu Lan View author publications You can also search for this
author inPubMed Google Scholar * An-Xin Wu View author publications You can also search for this author inPubMed Google Scholar CONTRIBUTIONS B.-C.T. discovered the reactions and conducted
the experiments; W.-X.L. carried out the DFT calculation. Y.L. and A.-X.W. directed the projects. X.-L.C, C.H, J.-T.M., and Y.-D.W. contributed to the discussion. CORRESPONDING AUTHORS
Correspondence to Yu Lan or An-Xin Wu. ETHICS DECLARATIONS COMPETING INTERESTS The authors declare no competing interests. ADDITIONAL INFORMATION PEER REVIEW INFORMATION _Nature
Communications_ thanks Junkai Fu and Per-Ola Norrby for their contribution to the peer review of this work. Peer reviewer reports are available. PUBLISHER’S NOTE Springer Nature remains
neutral with regard to jurisdictional claims in published maps and institutional affiliations. SUPPLEMENTARY INFORMATION SUPPLEMENTARY INFORMATION PEER REVIEW FILE DESCRIPTION OF ADDITIONAL
SUPPLEMENTARY FILES SUPPLEMENTARY DATA 1 RIGHTS AND PERMISSIONS OPEN ACCESS This article is licensed under a Creative Commons Attribution 4.0 International License, which permits use,
sharing, adaptation, distribution and reproduction in any medium or format, as long as you give appropriate credit to the original author(s) and the source, provide a link to the Creative
Commons license, and indicate if changes were made. The images or other third party material in this article are included in the article’s Creative Commons license, unless indicated
otherwise in a credit line to the material. If material is not included in the article’s Creative Commons license and your intended use is not permitted by statutory regulation or exceeds
the permitted use, you will need to obtain permission directly from the copyright holder. To view a copy of this license, visit http://creativecommons.org/licenses/by/4.0/. Reprints and
permissions ABOUT THIS ARTICLE CITE THIS ARTICLE Tang, BC., Lin, WX., Chen, XL. _et al._ Quadruple C-H activation coupled to hydrofunctionalization and C-H silylation/borylation enabled by
weakly coordinated palladium catalyst. _Nat Commun_ 11, 5662 (2020). https://doi.org/10.1038/s41467-020-19508-z Download citation * Received: 13 May 2020 * Accepted: 16 October 2020 *
Published: 09 November 2020 * DOI: https://doi.org/10.1038/s41467-020-19508-z SHARE THIS ARTICLE Anyone you share the following link with will be able to read this content: Get shareable
link Sorry, a shareable link is not currently available for this article. Copy to clipboard Provided by the Springer Nature SharedIt content-sharing initiative