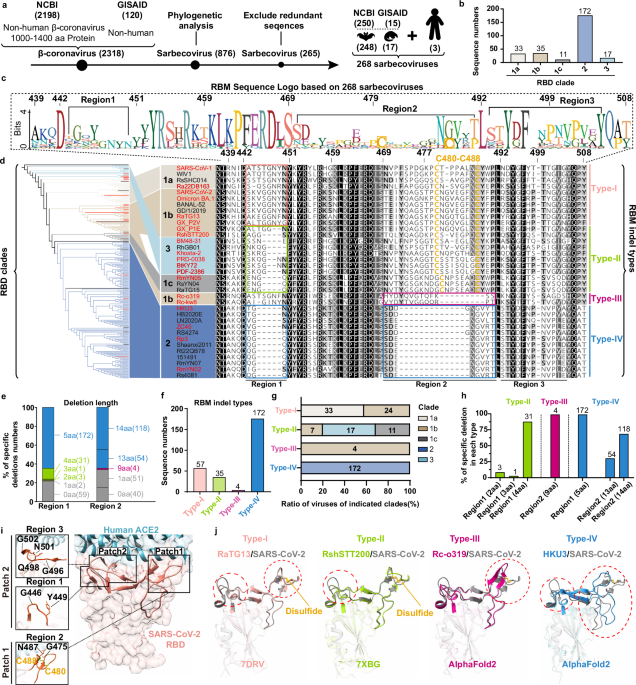
Sarbecovirus rbd indels and specific residues dictating multi-species ace2 adaptiveness
- Select a language for the TTS:
- UK English Female
- UK English Male
- US English Female
- US English Male
- Australian Female
- Australian Male
- Language selected: (auto detect) - EN
Play all audios:

ABSTRACT Our comprehensive understanding of the multi-species ACE2 adaptiveness of sarbecoviruses remains elusive, particularly for those with various receptor binding motif (RBM)
insertions/deletions (indels). Here, we analyzed RBM sequences from 268 sarbecoviruses categorized into four RBM indel types. We examined the ability of 20 representative sarbecovirus Spike
glycoproteins (S) and derivatives in utilizing ACE2 from various bats and several other mammalian species. We reveal that sarbecoviruses with long RBMs (type-I) can achieve broad ACE2
tropism, whereas viruses with single deletions in Region 1 (type-II) or Region 2 (type-III) exhibit narrower ACE2 tropism. Sarbecoviruses with double region deletions (type-IV) completely
lost ACE2 usage, which is restricted by clade-specific residues within and outside RBM. Lastly, we propose the evolution of sarbecovirus RBM indels and illustrate how loop lengths,
disulfide, and residue determinants shape multi-species ACE2 adaptiveness. This study provides profound insights into the mechanisms governing ACE2 usage and spillover risks of
sarbecoviruses. SIMILAR CONTENT BEING VIEWED BY OTHERS ACE2 BINDING IS AN ANCESTRAL AND EVOLVABLE TRAIT OF SARBECOVIRUSES Article Open access 03 February 2022 RECOMBINATION-AWARE
PHYLOGENETIC ANALYSIS SHEDS LIGHT ON THE EVOLUTIONARY ORIGIN OF SARS-COV-2 Article Open access 04 January 2024 SARS-COV-2 SPIKE PROTEIN PREDICTED TO FORM COMPLEXES WITH HOST RECEPTOR PROTEIN
ORTHOLOGUES FROM A BROAD RANGE OF MAMMALS Article Open access 05 October 2020 INTRODUCTION The Severe Acute Respiratory Syndrome (SARS) outbreak and COVID-19 pandemic significantly raised
global awareness of the zoonotic risks posed by sarbecoviruses1,2. The _Sarbecovirus_ subgenus, also known as lineage B β-coronaviruses, encompasses hundreds of SARS-related coronaviruses
exhibiting varying RBM sequences3,4,5,6,7,8,9,10,11,12,13,14. Most sarbecoviruses naturally infect Rhinolophus (horseshoe) bats, the primary natural reservoir15,16,17. Additionally,
sarbecoviruses sharing high receptor binding domain (RBD) similarity to SARS-CoV-2 have been identified in pangolins, such as GX/P2V/2017 (GX_P2V), GD/1/2019, and GX_P1E10,18. Sarbecoviruses
exhibit extensive genetic diversity in RBM, likely arising from frequent recombination and the high selective pressure associated with inter-species host jumping in bats and pangolins,
underscoring the risks of the emergence and outbreak of new human sarbecovirus19,20,21,22,23. However, many sarbecoviruses are known only as viral sequences and their ability to jump species
and spillover to humans remains unclear. Although ACE2 has been documented as a receptor for selected groups of setracovirus (e.g., NL63) and merbecoviruses (e.g., NeoCoV)24,25, it has been
primarily studied as the receptor for sarbecoviruses1,9,24. Notably, not all sarbecoviruses have been confirmed to use ACE2 as their receptor, especially RBD clade 2 sarbecoviruses, which
are proposed to utilize a distinct (yet unknown) receptor3,4,15. Nevertheless, ACE2 usage has been demonstrated in most representative sarbecoviruses other than clade 2
sarbecoviruses3,6,7,16,22,26. Structural analysis of ACE2 in complex with RBDs from various sarbecoviruses reveals a similar interaction mode, albeit with variations in specific residues
involved in recognition20,22,27,28. Specifically, the bridge-shaped RBM spanning amino acids (aa) 439-508 of SARS-CoV-2, formed by an extended loop connecting two β strands of the RBD core
subdomain and stabilized with disulfide-bridging, interacts with ACE2 at two distinct patches29,30. The interface on ACE2 mainly comprises the amino-terminal (N-terminal) α1 helix, along
with limited interactions with the α2 helix and a loop connecting the β3 and β4 strands29. Given the importance of receptor recognition in determining host barriers, assessing multi-species
ACE2 tropism for sarbecoviruses with distinct RBM features is crucial for understanding their zoonotic potential31,32. Previous studies have provided substantial insight into distinct
receptor preferences among bats and other mammalian species for SARS-CoV-1, SARS-CoV-2, GX_P2V, RaTG13, NeoCoV, and others20,22,25,33,34,35,36,37,38,39,40,41,42. Varying entry-supporting
abilities have also been observed in ACE2 orthologues from the same bat species but with different polymorphisms, particularly in residues involved in sarbecovirus
binding21,43,44,45,46,47,48. Sarbecoviruses are commonly classified into several clades based on the RBD phylogeny and ACE2 usage3,4,23. Despite sharing a similar RBD core subdomain,
sarbecoviruses exhibit diversity in RBM sequences, particularly the presence of various indels in Region 1 (residues 443-450SARS-CoV-2) or Region 2 (residues 470-491SARS-CoV-2)4,23. Clade 1
sarbecoviruses are all ACE2-using sarbecoviruses which can be further divided into subclades 1a, 1b, and 1c based on RBD phylogeny3. Most clade 1a (SARS-CoV-1 lineage) and 1b (SARS-CoV-2
lineage) sarbecoviruses have the longest RBM and lack RBM deletions of more than one amino acid3. Several sarbecoviruses with Region 1 or Region 2 single RBM deletions that are identified as
clade 1b viruses, such as RshSTT182, RshSTT20049, Rc-o319, and Rc-kw85, which were recently found in Cambodia and Japan, respectively. Clade 1c sarbecoviruses include a subgroup of recently
reported Asian sarbecoviruses carrying single Region 1 deletion, such as RmYN05, RaTG15, and RsYN04, which are also known as clade 4 sarbecoviruses in some studies6,7,50. Here, we described
these sarbecoviruses as 1c subclade considering their RBD phylogeny, geographical distribution, and ACE2 usage compared with 1a and 1b. Clade 2 sarbecoviruses are phylogenetically close to
clade 1 and characterized by the two deletions (or indels) within RBM3,4,15,16,32,51,52,53. Clade 3 sarbecoviruses, such as BM48-31, Khosta-1/2, BtKY72, PDF-2386, and PRD-0038, discovered in
Africa and Europe are considered closer to the sarbecovirus ancestors and all carry single deletions (indels) in RBM Region 13,9,14,54,55,56,57. Several clade 3 sarbecoviruses have been
demonstrated as ACE2-using viruses, supporting this receptor usage as an ancestral trait of sarbecoviruses3,28,51,58. Although proposed to have evolved from ACE2-using ancestors through the
subsequent receptor switch3,54, whether all clade 2 sarbecoviruses have lost ACE2 usage across all ACE2 orthologues remains an open question. Our understanding of the key determinants
affecting sarbecoviruses multi-species ACE2 adaptiveness and the factors restricting ACE2 usage remains incomplete. With an increasing number of sarbecoviruses identified with various single
RBM indels, addressing the impact of these indels on multi-species ACE2 tropism becomes crucial. Moreover, sarbecoviruses with similar RBM deletion patterns exhibit marked differences in
ACE2 tropism profile, emphasizing the role of critical RBD residues impacting multi-species ACE2 recognition beyond loop deletions20,21,45,51. In this study, we analyze the 268 sarbecovirus
S sequences to delineate the overall RBM indel features and categorize them into four RBM indel types. Employing an ACE2 library consisting of 56 orthologues, we extensively evaluate
cellular RBD binding and pseudovirus entry of 20 representative sarbecoviruses and various derivatives, encompassing RBM loop chimera and mutations. We elucidate multiple key determinants
for ACE2 recognition and show how loop lengths, disulfide, and restrictive residues dictate ACE2 tropism and adaptiveness to ACE2 orthologues from various species. Our data lead to a more
comprehensive understanding of the multi-species ACE2 adaptiveness across sarbecoviruses, as well as the coevolution of RBM indels and ACE2 adaptiveness. RESULTS FOUR RBM INDEL TYPES FOR
SARBECOVIRUSES We retrieved 2318 Non-human β-coronavirus S glycoprotein sequences from the NCBI and GISAID databases, with 876 distinguished as sarbecovirus based on phylogenetic analysis.
After reducing redundancy by excluding identical sequences and those highly similar to SARS-CoV-1 and SARS-CoV-2 (>99% identity), we obtained 265 sarbecovirus S sequences for further
investigation, with 17 from pangolins, 1 Hipposideros bat and 247 Rhinolophus bats. We also included 3 representative human sarbecovirus sequences (SARS-CoV-1, SARS-CoV-2-WT, and
SARS-CoV-2-Omicron BA.1) for analysis (Fig. 1a and Supplementary Data 1). Phylogenetic analysis based on RBD protein sequences revealed five sub-clades (RBD clades 1a, 1b, 1c, 2, and 3),
with clade 2 accounting for the largest (Fig. 1b, Supplementary Fig. 1a). Multi-sequence alignment and Sequence Logo analysis highlighted three highly variable regions in RBMs, with Regions
1 and 2, but not Region 3, being the hot spots of loop indels (Fig. 1c, and Supplementary Data 2). For the five subclades, RBM sequences of 35 representative sarbecoviruses from each main
phylogenetic branch were selected to demonstrate the diversity of RBM sequences. The selection criteria include RBD phylogeny, RBM sequence similarity, indel types, indel lengths, and
preference for sequences with cryo-EM structure availability (Fig. 1d, and Supplementary Fig. 1a–d). To better investigate the impact of RBM indels on multi-species ACE2 adaptiveness, we
categorized sarbecoviruses into four RBM indel types in addition to the clade-based classification. Specifically, RBM type-I describes most clade 1a and 1b sarbecoviruses without RBM
deletions relative to SARS-CoV-1 or SARS-CoV-2 and are considered RBM prototypes, RBM type-II and type-III are viruses with single RBM deletions in Region 1 or Region 2, respectively, RBM
type-IV viruses correspond to clade 2 sarbecoviruses with dual RBM deletions (Fig. 1d). In analyses of RBM deletions of the 268 sequences, it was found that deletions of 1, 2, 3, 4, or 5
amino acids (aa) can occur in Region 1, and deletions of 1, 9, 13, or 14 aa can occur in Region 2 (Fig. 1e). Notably, the 5aa deletion in Region 1 is always accompanied by a 13 or 14 aa
deletion in Region 2. Accordingly, four RBM types were defined based on the presence of deletions (>1aa) in Region 1 (type-II), Region 2 (type-III), or both (type-IV), while sequences
with no deletion or only 1aa deletion were classified as type-I. This classification led to different subgroups of sarbecovirus (RBM types) compared to the clades based on RBD phylogeny
(Fig. 1f–g). For example, all clade 1a sarbecoviruses (SARS-CoV-1 lineage) are RBM type-I, all clade 3 and clade 1c sarbecoviruses are RBM type-II, while clade 1b (SARS-CoV-2 lineage)
sarbecoviruses can be divided into RBM type-I, II, and III (Fig. 1g). The deleted amino acid numbers in Region 1 and 2 of different sarbecoviruses are summarized based on different RBM types
(Fig. 1h). After reducing redundancy by removing sarbecoviruses with high RBM sequence similarity, 20 representative sarbecoviruses were selected for subsequent functional profiling of
multi-species ACE2 tropism, encompassing sarbecoviruses from all five subclades and covering all kinds of deleted numbers in either Region 1 or Region 2 (Supplementary Fig. 1b, c). Amino
acid sequence identity analyses revealed that these sarbecoviruses share at least 57.07% RBD identity and 21.54% RBM identity in amino acid sequences, suggesting greater genetic variation in
RBM than in RBD (Fig. 1d and Supplementary Fig. 1d). From a structural perspective, the spatially proximate Region 1 and Region 3 loops form interaction patch 2, while the majority of
residues in Region 2 loop contribute to interaction patch 1 (Fig. 1i). Interestingly, the conserved disulfide bridge for stabilizing loop in Region 2 is absent in RBM type-III and IV
sarbecoviruses (Fig. 1d, i)59. Superimposition of the solved or AlphaFold2-predicted RBDs with that of SARS-CoV-2 highlighted shortened extended loops due to specific deletions (Fig. 1j).
Given that the two deletions are situated in critical RBM extensions for ACE2 interaction, their presence might impact multi-species ACE2 tropism. We utilized a well-established
RBD-hFc-based assay to assess the live cell receptor binding (Supplementary Fig. 2a–c). Due to the unavailability of the authentic sarbecovirus strains, we employed a dual reporter-based
vesicular stomatitis virus (VSV) pseudotyping system assembled with sarbecovirus S glycoproteins to assess receptor functionality of various ACE2 orthologues (Supplementary Fig. 2d–f)4. The
S glycoproteins from these sarbecoviruses incorporated into the pseudovirus particles were adjusted at comparable levels for entry assays (Supplementary Fig. 2g). The two different
functional assays provide cross-validation and migrate the potential impact of other S components on viral entry efficiencies, such as NTD and S2. MULTI-SPECIES ACE2 USAGE PROFILE To
illustrate a comprehensive ACE2 usage spectrum of each sarbecovirus, we examined 56 ACE2 orthologues from 51 bat and 5 representative non-bat mammalian species (Supplementary Fig. 3a, b).
The bat species represent a broad genetic diversity spanning 11 bat families with global distribution and genetic diversity, including eight Rhinolophus bats geographically across Europe,
Africa, and Asia (Fig. 2a, and Supplementary Fig. 3c)33. Sequence analysis of these ACE2 orthologues exhibited significant variations in residues potentially involved in sarbecovirus
interactions (Supplementary Fig. 3a, b). HEK293T cells stably expressing ACE2 orthologues were established and maintained with verified expression33 (Supplementary Fig. 4). RBD binding
assays based on the 20 selected sarbecoviruses with distinct RBM features were conducted to investigate their multi-species ACE2 binding spectra (Fig. 2a). According to the binding data, a
subset of 17 sarbecoviruses were tested with the pseudovirus entry assays to evaluate their ability to utilize different ACE2 orthologs for entry. (Fig. 2b). These two assays displayed
generally consistent ACE2 usage patterns, with a few exceptions. These apparent discrepancies in two different assays are commonly observed when testing other coronaviruses. Except for the
RBD concentrations or viral titer/infectivities that may result in differences in assay sensitivity, other factors also contribute to the discrepancies. For example, the multi-step entry
process is not always guaranteed by RBD binding alone, weak RBD binding can sometimes be sufficient for entry as the binding is a dynamic process and multivalent binding through spike
trimmers in pseudoviral particles can increase binding avidity. Except for the type-IV RBM sarbecoviruses, the other sarbecoviruses displayed confirmed ACE2 usage, albeit with different ACE2
usage spectra. Several type-I RBM viruses, like SARS-CoV-1, SARS-CoV-2, and GX_P2V, efficiently use most orthologues, including human ACE2 (hACE2) (Fig. 2a). In contrast, RBM type-II or
type-III sarbecoviruses generally showed narrower ACE2 tropism, and most are unable to use hACE2, representing a relatively low ACE2 adaptiveness to achieve broad ACE2 tropism compared to
RBM type-I sarbecoviruses. Notably, GX_P1E, an RBM type-II pangolin sarbecovirus closely related to GX_P2V, with only 2aa (NY) deletion in RBM Region 1 displayed narrower ACE2 tropism,
suggesting that 2aa reduction in Region 1 length is sufficient to affect the breadth of multi-species ACE2 tropism. Although PRD-0038 has been proposed as a sarbecovirus with broad ACE2
tropism among Rhinolophus bats28, this virus, along with four other clade 3 sarbecoviruses (Khosta-2, BtKY72, PDF-2386, and BM48-31) with 2 to 4aa deletions in Region 1, display a narrow or
moderate breadth of ACE2 tropism in our study (Fig. 2a). The RBD binding of the five sarbecoviruses to their optimal ACE2 orthologues, mostly from their hosts, was further analyzed through
flow cytometry and Bio-layer interferometry (BLI) (Fig. 2c, d). Notably, two close-related RBM type-I sarbecoviruses with identical RBM lengths, GX_P2V and RaTG13, displayed contrasting
breadth of ACE2 tropism (Fig. 2a, b). We hypothesize that the narrow tropism of RaTG13 could be attributed to suboptimal residues restricting its binding with ACE2 orthologues. Supporting
this hypothesis, the previously reported RaTG13 mutations, T372A43,47,60 (removing a glycan at N370) and T403R/K45,46 (enabling additional ACE2 interaction), significantly expanded the
spectrum of ACE2 usage in our study (Supplementary Fig. 5a, b). Furthermore, analysis based on eight swap mutants exchanging RBM residues on 493, 498, 501, and 505 (SARS-CoV-2 numbering)
reveals point mutation at position 501, in addition to position 372 and 403, can also markedly expand the breadth of ACE2 tropism of RaTG13, suggesting a relatively high muli-species ACE2
adaptiveness of this virus (Fig. 2e–h, Supplementary Fig. 5c)3,43,61,62. To investigate the mechanism of restrictive effect of D501, residue usage analysis was conducted on six ACE2
positions spatially close to position 501, which revealed an overall negatively charged surface among the 56 orthologues, thereby disfavoring D501 due to electrostatic repulsion (Fig. 2i).
This hypothesis is further supported by similar phenotypes of RshSTT200, SARS-CoV-1, and SARS-CoV-2 carrying D/T mutations at this position (Supplementary Fig. 5d,e and Supplementary Fig.
6a–d). Since the N501Y mutation became dominant during the spread of SARS-CoV-2 in humans, we also compared the multi-species ACE2 usage spectra of SARS-CoV-1 and SARS-CoV-2 carrying N or Y
at position 501SARS-CoV-263. The Y mutation at this position slightly increased the number of acceptable ACE2 orthologues for SARS-CoV-2 while dramatically reducing acceptable ACE2 to only
four orthologues for SARS-CoV-1 (Supplementary Fig. 6a–d). Structural analysis suggests Y487SARS-CoV-1 may cause steric hindrance with local Y41hACE2 and K353hACE2, whereas the
Y501SARS-CoV-2 instead forms a π-π stacking interaction with Y41hACE2 and cation-π interaction with K353. This indicates the virus-specific phenotypes can be attributed to the structural
discrepancies of residues at equivalent positions (Supplementary Fig. 6e)64. Similarly, the 501 T mutations in RmYN05 or Rc-o319 have no significant impact on their breadth of ACE2 tropism
(Supplementary Fig. 5d, e). Moreover, the different efficiencies of SARS-CoV-2, SARS-CoV-2-N501Y, and SARS-CoV-2-Omicron BA.1 in using various ACE2 orthologues indicated the presence of
residues, other than the 501 residues, affecting the ACE2 tropism of Omicron BA.1, a phenotype that also observed in authentic SARS-CoV-2 infection assays (Supplementary Fig. 7a, b). Fine
mapping of the mutations in BA.1 underscores the critical contribution of residues at position 493 in affecting multi-species ACE2 tropism (Supplementary Fig. 7c–g). Collectively, these data
indicated that the RBM type-I sarbecoviruses exhibited superior multi-species ACE2 adaptiveness among the tested 20 sarbecoviruses, although the tropism breadth can be modulated by critical
residues within or near the interaction surface, such as residues at positions 403, 501, and 493 (SARS-CoV-2 numbering). THE IMPACT OF RBM INDELS ON ACE2 TROPISM To investigate the impact
of loop lengths on multi-species ACE2 tropism, we generated chimeras with specific loop substitutions in Region 1 or Region 2. These include SARS-CoV-2 with single deletions in each region
and other sarbecoviruses carrying partial or entire loop substitutions with SARS-CoV-2 equivalent sequences (Fig. 3a). The VSV packaging efficiency of all S chimeras was validated by Western
blot (Fig. 3b). SARS-CoV-2 with a KVNY deletion in Region 1 (ΔRegion1*, relative to RshSTT200) displayed reduced multi-species ACE2 tropism but still can use ACE2 from a subset of species,
including humans (Fig. 3c, d). However, the 9aa deletion in Region 2 (ΔRegion2*, relative to Rc-o319) abolished its ability to use any tested ACE2 orthologues. BM48-31 and Rc-o319 with
regions substituted by SARS-CoV-2 equivalents can use more ACE2 orthologues, yet remain unable to achieve a broad tropism as RBM type-I sarbecoviruses. For RshSTT200 and Rc-o319, the
increase of multi-species ACE2 tropism was not achieved by filling the deletions unless the entire RBM region was substituted with SARS-CoV-2 counterparts. This indicates the importance of
side chains or local conformations apart from loop lengths (Fig. 3c, d). Notably, the highly conserved RBM disulfide bridge is present in Rc-o319-R2 but absent in Rc-o319-R2*, the importance
of which was confirmed by the loss of infectivity of SARS-CoV-2 C480S and Rc-o319-R2 C462S mutants in using all tested ACE2 orthologues59,65. However, introducing a disulfide to Rc-o319-R2*
via K454C mutation remains insufficient to restore its ability to use ACE2, suggesting the presence of incompatible residues for Region 2-ACE2 interaction (Fig. 3e–h). Substituting both R1
and R2 regions, which also introduced the featured disulfide, in the three RBM type-IV (clade 2) sarbecoviruses (ZC45, RmYN02, HKU3) failed to achieve any detectable ACE2 usage signal in
both binding and pseudovirus entry assays (Fig. 3c, d). This indicates the presence of determinants other than loop deletions that restrict ACE2 usage in RBM type-IV sarbecoviruses4. CLADE
2-SPECIFIC RESIDUES RESTRICTING ACE2 USAGE In earlier attempts to identify determinants restrict ACE2 usage for clade 2 sarbecoviruses, we unexpectedly found that HKU3 and ZC45 remained
unable to bind any ACE2 even with the entire RBM (aa439-508) replaced with SARS-CoV-2 RBM, indicating the presence of determinants restricting ACE2 recognition outside the RBM (Supplementary
Fig. 8a). When comparing RBD sequences from 172 RBM type-IV (clade 2) sarbecoviruses with the other 96 ACE2-using sarbecoviruses, 22 clade 2-specific residues situated within or outside the
RBMs were identified (Fig. 4a). It has been proposed that two residues (D496 and P502) within the Region 3 of RBM type-IV sarbecoviruses may restrict potential ACE2 interaction based on
structural modeling, while the impact of these two residues and other clade 2-specific residues outside RBM on ACE2 recognition remains to be investigated using cell-based functional
assays66. To identify the determinants restricting ACE2 recognition, we conducted chimeric HKU3 S mutants with corresponding RBD sequences substituted with SARS-CoV-2 equivalents. HKU3 was
selected as it represents the clade 2 sarbecovirus showing the highest SARS-CoV-2 RBD amino acid sequence identity (63.92%) in our study (Supplementary Fig. 1d). Sequence alignment of
SARS-CoV-1, SARS-CoV-2 and HKU3 RBD displayed 16 HKU3-specific residues upstream of the RBM region (Fig. 4b). The subsequent functional dissection initiated from large fragment swaps and
then proceeded to fine mapping of single residue combinations (Fig. 4b, c). In addition to SARS-CoV-2 RBM replacement (HKU3-RBMSARS2), further substituting fragment A (aa 385-417) enabled
HKU3 to use hACE2 for entry but remained deficient in binding hACE2 efficiently. Extending by fragment B (aa354-417) and fragment C (aa349-417) underscore the critical contribution of
S349SARS-CoV-2 for efficient ACE2 binding (Fig. 4d, e). Fine mapping of fragment A highlighted the crucial role of six clade 2-specific residues at positions 388, 394, 399, 401, 404, 405
(SARS-CoV-2 numbering) that restricting hACE2 usage, with S349 + V401G404D405 (S + VGD) being the minimal combination (Fig. 4c–e and Supplementary Fig. 8b). Similar results were obtained
when testing two other clade 2 (RBM type-IV) sarbecoviruses, ZC45 and RmYN02 (Supplementary Fig. 8b, d–h). The expanded multi-species ACE2 usage spectra of HKU3-RBMSARS2 carrying S + NNSVGD
or S + VGD mutations were demonstrated by RBD binding and pseudovirus entry assays (Fig. 4f, g). Furthermore, the ACE2 dependence of HKU3 S mutant (HKU3-RBM S + NNSVGD) was verified by the
efficient amplification of a propagation-competent recombinant VSV genetically encoding the S mutant in Caco2-hACE2 cells, which could be dose-dependently neutralized by the hACE2-specific
antibody h11B11 (Fig. 4h and Supplementary Fig. 8c)67. The restrictive effect of these clade 2-specific residues was further demonstrated by the loss of ACE2 usage of SARS-CoV-2 carrying
mutations at equivalent positions. SARS-CoV-2 carrying the corresponding mutants within or outside the RBM region (S349N, V401L, V401L + G404S + D405S, G496D + G502P, and P507A + Y508T) all
underwent a significantly reduced efficiency in using hACE2 (Fig. 4i, j). The loss of hACE2 binding affinity of SARS-CoV-2 RBD harboring S349N or V401L point mutations was further verified
by BLI assays (Fig. 4k). The restrictive effect of residues at these two positions can also observed in RmYN02 (clades 2) and SARS-CoV-1 (clade 1a), but is less pronounced in BM48-31 (clade
3) with higher RBD sequence divergence (Supplementary Fig. 8d–j). Interestingly, unlike R403SARS-CoV-2 which directly interacts with ACE2, the two clade 2-specific residues crucial for ACE2
binding, S349SARS-CoV-2 and V401SARS-CoV-2, are situated underneath the canonical RBM. Thus, S349N and V401L mutations (SARS-CoV-2 numbering) in HKU3 may slightly alter the RBM conformation
due to their relatively larger side chains (Supplementary Fig. 8i). The resulting conformational shift may lead to the mismatch of critical residues for ACE2 interaction, thereby restricting
ACE2 usage of clade 2 sarbecoviruses even when the entire RBM region is replaced by SARS-CoV-2 counterparts. COEVOLUTION OF RBM INDELS AND ACE2 ADAPTIVENESS To trace the coevolution of
sarbecoviruses RBM indels and ACE2 adaptation, we integrated biological functional data on multi-species ACE2 tropism with in silico analyses based on RBD clades, RBM types, and residue
usages in the two indel-containing regions (Fig. 5a–c and Supplementary Fig. 9a, b). The breadth of multi-species ACE2 tropism and hACE2 fitness jointly affect the overall spillover risks of
sarbecoviruses of different clades and RBM indel types (Fig. 5a, b). The scrutiny of the sequence features reveals an intriguing indel pattern in Region 1, characterized by one or two
conserved and centrally located glycine (G), while Region 2 displays a complex indel rather than straightforward 9aa or 13/14aa deletions in RBM type-III and IV sarbecoviruses, respectively
(Fig. 5c and Supplementary Fig. 9b). Notably, a potential evolutionary trace of Region 1 insertion was identified by the likely duplication of NY/NF sequences on the right side of the G
(Fig. 5c). Combining these data, we proposed an evolutionary pathway delineating the emergence of various RBM types, highlighted by critical events driving the evolution (Fig. 5d). While the
origin of the common ancestor of sarbecoviruses remains elusive, Africa/Europe sarbecoviruses (clade 3) maintained a relatively ancient state of RBM indel type-II3. The Asia sarbecoviruses
underwent extensive evolution and developed into clade 1 and 2 sarbecoviruses with significant genetic diversity. These viruses evolved in three different directions with distinct ACE2
adaptiveness. Clade 1c sarbecoviruses maintained RBM type-II with limited genetic diversities based on currently known sequences. Clade 2 sarbecoviruses underwent R1 (-5aa) and R2 (-13/14aa)
deletions (or indels) and switched to a non-ACE2 receptor, coupled with the emergence of clade 2-specific residues that further restricted ACE2 usage. In contrast, clade 1a and 1b viruses
underwent Region 1 insertion (or indels with increased residue numbers), generating the longest (8aa) Region 1 for underpinning the interactions, thereby achieving the highest multi-species
ACE2 adaptiveness. Some clade 1b viruses may have undergone further or different indels events in Region 1 (-2 to -4aa) and Region 2 (-9aa), resulting in RBM type-II (e.g. RshSTT200 and
GX_P1E) and type-III (e.g. Rc-o319 and Rc-kw8), respectively, with moderate multi-species ACE2 adaptiveness. (Fig. 5d, e). While Region 1 is shorter than Region 2, it exhibits more diverse
sequence changes in ACE2-dependent sarbecoviruses, fine-tuning the species-specific ACE2 adaptiveness. By contrast, no further RBM indel change was observed among all 172 clade 2
sarbecoviruses. Intriguingly, despite high sequence variability in Region 1, only two out of the 268 sequences, BM48-31 and BB9904, lack a G in this region. In RBM type-I sarbecoviruses with
8aa length, most clade 1b viruses have two conserved G (2 G), whereas most 1a viruses have an SG or TG (Fig. 5c, e). From a structural aspect, indels in Region 1 resulted in different loop
lengths, with a G close to the turn of the loop conferring flexibility. The elongated Region 1 loop allows closer distance and potential H-bond formation with ACE2, reinforcing the patch 2
ACE2 interaction network along with the Region 3 loop, which explains the superior multi-species ACE2 adaptiveness of RBM type-I sarbecoviruses (Fig. 5f). The importance of residue usage in
Region 1 is supported by the data showing a dramatic decrease in multi-species ACE2 usage in SARS-CoV-2 carrying a G447Y mutation (Fig. 5g and Supplementary Fig. 10a–c). Additionally, we
observed a reduced multi-species ACE2 adaptiveness of the “G-free” 4aa deletion mutant, SARS-CoV-2-ΔGGNY (Region 1: DSKVNY), compared to SARS-CoV-2-ΔKVNY (Region 1: DSGGNY) (as shown in Fig.
3), the former only recognize R.alc ACE2, similar to the phenotype of BM48-31 which also lacks G in region 1 (Fig. 5g and Fig. 2a, b). SARS-CoV-2-ΔGGNY may employ a similar ACE2 recognition
mode as BM48-31, considering the importance of position 31 R.alcACE2 for both viruses in a swap mutagenesis experiment based on R.alc ACE2 and its closely related orthologue, R.fer ACE2
(Supplementary Fig. 11a–d). This suggests that Region 1 deletion might be more tolerable for binding with multiple ACE2 orthologues if smaller side chains, like G, are maintained in this
region. DISCUSSION The persistent evolution of sarbecoviruses in Rhinolophus bats drives the emergence of sarbecovirus clades with varying RBM sequences. Frequent sequence changes,
particularly indels within the RBM, pose challenges in predicting the potential of sarbecoviruses to cross species barriers and spillover to humans. To better investigate the influence of
indels and other determinants on multi-species ACE2 usage, we propose a new RBM indel-based classification, categorizing all currently identified sarbecoviruses into four distinct RBM indel
types. Our functional data, combined with extensive sequence analyses, provide a comprehensive profile of the multi-species ACE2 tropism of sarbecoviruses belonging to specific clades and
RBM indel types (Supplementary Fig. 12). Despite narrower ACE2 tropism, all tested sarbecoviruses carrying single RBM deletion in either Region 1 or Region 2 exhibited confirmed ACE2 usage,
typically adapted to ACE2 from their hosts. Furthermore, we hypothesize an evolutionary history regarding the emergence of sarbecoviruses with distinct RBM indel types. As the number of
sarbecovirus sequences from different clades increases, the intricate evolutionary history of sarbecoviruses remains to be updated or amended. The driving force behind the emergence of
different sarbecovirus Region 1 and Region 2 indels remains elusive. Virus recombination may play a crucial role, as the RBM or even Region 1 has been predicted as a breaking point for
combinations between sarbecoviruses54. Although various NTD-indels emerged in SARS-CoV-2 during the pandemic, no indels have been detected in RBM Region 1 or Region 2 in prevalent variants,
suggesting a different evolution mechanism of RBM indels formation of various sarbecoviruses in bats compared to SARS-CoV-2 in humans68. Our results reveal a coevolution between sarbecovirus
indels and multi-species ACE2 adaptiveness. Remarkably, the fine-tuning of RBM Region 1 through various indels and specific side chains promotes the emergence of sarbecoviruses with
distinct multi-species ACE2 usage spectra. This could be attributed to the dispensable trait of Region 1 for interaction with a specific ACE2 orthologue (including hACE2), compensated by the
Region 3 loop without indels. Additional interactions mediated by the extended Region 1 loop in RBM type-I sarbecoviruses might be crucial for achieving broader host tropism and
facilitating host jumping. Interestingly, a conserved G within Region 1 suggests that greater flexibility or the absence of a large side chain in this site may confer certain evolutionary
advantages. Comparatively, indels in Region 2 are less diverse than in Region 1 and generally have a more dramatic impact on ACE2 recognition, or even result in a receptor switch to a
yet-unknown receptor. It is worth noting that limited multi-species ACE2 adaptiveness may restrict viral host jumping ability. However, this does not preclude the ability to use or adapt to
hACE2, as is exemplified in Khosta-2 or other clade 1b or 3 sarbecovirus mutants described in this and prior studies3,28,45,57,58. Filling RBM deletions with SARS-CoV-2 counterparts does not
guarantee a broader ACE2 usage spectrum and may sometimes result in reduced or lost ACE2 usage. This underscores the enhanced ACE2 adaptiveness achieved during adaptive evolution, with both
length and residues being optimized in specific indels. Consequently, substituting the entire loop sometimes is necessary for achieving higher ACE2 compatibility. However, RBM type-IV
sarbecoviruses, even after gap-filling or entire RBM substitution, remained unable to use any ACE2 orthologues, which led to the identification of clade-specific determinants outside the RBM
that restrict ACE2 usage, likely due to adaptation to another receptor usage. Some critical RBD core residues are underneath the RBM, indirectly restricting ACE2 binding by affecting the
RBM conformation. Future structural analysis could elucidate how these determinants affect receptor recognition. A main limitation of this study is our analyses are based on the publicly
available sarbecovirus sequences which may not recapitulate all authentic sarbecoviruses in nature. There could be additional RBM indel types yet to be discovered and evaluated. Although we
tried to include most S glycoprotein sequences with distinct features, there might still be exceptions. For example, certain RBM type-II sarbecoviruses not tested might achieve a broad ACE2
tropism through compensatory interactions not contributed by RBM region 1. Moreover, we acknowledge that it is insufficient to predict tropism patterns solely based on RBD clades and RBM
indel types, as the pattern can be affected by single restriction residues. Considering the current data of restrictive clade 2-specific RBD core residues are based on RBM type IV chimeras
with SARS-CoV-2 RBM, other ways of acquiring ACE2 binding may exist for these viruses harboring different RBM sequences. It should also be noted that although ACE2 compatibility is a primary
barrier for sarbecoviruses to cross species, efficient ACE2 recognition alone does not guarantee susceptibility at the animal level. Other factors, such as host protease, immune response,
and viral replication efficiency, also affect host tropism, which can be verified by authentic viruses and in vivo studies in the future50,69,70. In conclusion, our RBM indel-type
classification offers a more precise way to describe sarbecoviruses when combined with RBD phylogenetic information. Our functional ACE2 usage data elucidate the mechanism governing
multi-species ACE2 usage and adaptiveness, shaped by multiple factors such as the presence and features of RBM loop deletions, RBM disulfide bridges, critical RBM residues for direct
interaction, and restrictive residues within and outside the RBM. These findings establish a solid scientific foundation for risk assessment and viral surveillance to mitigate potential
future zoonoses caused by these viruses. METHODS CELL CULTURE BHK-21 (ATCC, CCL-10), Caco2 (ATCC, HTB-37), HEK293T (ATCC, CRL-1586) cells and their derivatives were maintained in Dulbecco’s
modified eagle medium (DMEM; Gibco) supplemented with 10% fetal bovine serum (FBS), 2.0 mM L-Glutamine, 110 mg/L sodium pyruvate, and 4.5 g/L D-glucose. I1-Hybridoma (CRL-2700), secreting a
monoclonal antibody targeting VSV glycoprotein (VSV-G), was maintained in Minimum Essential Medium with Earle’s salts and 2.0 mM L-Glutamine (MEM; Gibco). All cells were cultured at 37°C in
5% CO2 with regular passage every 2-3 days. GENE SEQUENCES Sarbecovirus S glycoprotein sequences are retrieved from NCBI Virus and GISAID databases. The keywords used for sequence search
include “Betacoronavirus”, “Sequence length between 1000-1400” “protein” and “NOT Homo sapiens” for NCBI and “bat”, “pangolin”, “civet”, coronaviruses for GISAID. A comprehensive collection
of 2,318 Betacoronavirus S glycoprotein sequences was obtained. After extracting 876 sarbecovirus sequences through phylogenetic analysis using Geneious, the dataset was refined to 265
unique sequences for further analysis by excluding redundant entries. The ACE2 orthologues sequences were summarized by previous reports33. Several additional ACE2 orthologues tested in this
study include _Rhinolophine Malayanus_ (Provided by Professor Huanbin Zhao, Wuhan University, China), _Rhinolophus shameli_ (GenBank: MZ851782), _Rhinolophus cornutus_ (GenBank:
BCG67443.1), _Rhinolophus sinicus isolate Rs-3357_(GenBank: KC881004.1), _Rhinolophus affinis_ (GenBank: QMQ39227.1), _Manis javanica (Pangolin)_(GenBank: XP_017505752.1), _Mouse_ (GenBank:
NP_001123985), _Camelus_ (GenBank: XP_006194263), _Civet_ (GenBank: Q56NL1), and _Rhinolophus alcyone_ (GenBank: ALJ94035.1). Human Aminopeptidase N precursor (APN) (GenBank: NP_001141.2)
was included as a negative control. The sources and accession numbers of the receptors and the 268 sarbecoviruses were summarized in Supplementary Data 1. BIOINFORMATIC ANALYSIS Amino acid
or nucleotide sequences from viruses or ACE2 orthologues were aligned using Mafft v7.45071. Phylogenetic trees were generated with IQ-Tree (version 2.0.6)72 using a Maximum Likelihood model
with 1000 bootstrap replicates. Tree annotations were performed using iTOL (https://itol.embl.de/). Sequence identities were analyzed by Geneious Prime (https://www.geneious.com/) after
being aligned by Mafft. The residue usage frequency (Sequence Logo analysis) was generated by the Geneious Prime. PLASMIDS The coding sequences of various coronavirus S glycoproteins and
their derivatives were human codon optimized and cloned into the pCAGGS vector with C-terminal 18-amino acids replaced with an HA tag (YPYDVPDYA) for improving VSV pseudotyping efficiency
and enabling detection73,74. The plasmids for expressing ACE2 orthologues are constructed by inserting human codon-optimized ACE2 sequences into a lentiviral transfer vector (pLVX-IRES-puro)
with a C-terminal 3×FLAG-tag (DYKDHD-G-DYKDHD-I-DYKDDDDK) for detection. The plasmids expressing the recombinant coronaviruses RBD human IgG Fc (RBD-hFc) fusion proteins were constructed by
inserting RBD sequences corresponding to SARS-CoV-2 (aa331−524) containing an N-terminal CD5 secretion signal peptide (MPMGSLQPLATLYLLGMLVASVL) and C-terminal hFc-twin-strep tandem tags for
purification and detection. ACE2 STABLE CELL LINES ACE2 expressing stable cell lines were established by lentiviral transduction33,75. Briefly, lentivirus carrying the ACE2 genes was
generated by co-transfecting pLVX-IRES-puro-ACE2 orthologues, pMD2G (plasmid no. 12259; Addgene), and psPAX2 (plasmid no. 12260; Addgene) into HEK293T cells. HEK293T or Caco2 cells were
subsequently transduced with the lentiviruses, and the stable cells expressing ACE2 orthologues were selected in the presence of puromycin (1 μg/mL). The expression levels of ACE2
orthologues were assessed using an immunofluorescence assay33. Briefly, HEK293T cells were fixed with 4% paraformaldehyde for 10 min at room temperature, permeabilized with 0.2% Triton
X-100/PBS for 10 min, and blocked with 1% BSA for 30 min at 37 °C. Subsequently, the cells were incubated with M2 antibody (anti-FLAG-tag, catalogue no. F1804A-5MG; Sigma) at 4 °C for 1
hour. After three washes with PBS, the cells were treated with 2 μg/mL Alexa Fluor 594-conjugated goat anti-mouse IgG (catalogue no. A11032; Thermo Fisher Scientific). Nucleus was stained
blue with Hoechst 33342 (1:5,000 dilution in PBS). Images were captured with a fluorescence microscope (MI52-N; Mshot). Relative fluorescence unit of Alexa Fluor 596 and Hoechst 33342 was
quantified by Thermo Varioskan LUX. The expression of most ACE2 orthologues was also verified by Western Blot analysis in our previous reports33. RECOMBINANT PROTEIN EXPRESSION AND
PURIFICATION Recombinant RBD-hFc fusion proteins or ACE2 ectodomains (amino acid sequences 18-740 correspond to Human ACE2) fused with FLAG-strep-tag proteins were generated through
transient transfection of HEK293T cells using Lipofectamine 2000. The transfected cells were cultured in SMM 293-TIS Expression Medium (Serum-free, without L-Glutamine) (Sino Biological).
The supernatant, containing the recombinant proteins, was collected at 2, 4, and 6 days post-transfection, and the expression was confirmed through Western Blot analysis using the Goat
Anti-Human IgG-Fc secondary Antibody (HRP) (SinoBiological Inc, SSA002) for RBD or the M2 antibody for ACE2. Protein purification was performed using Protein A/G Plus Agarose (Thermo Fisher
Scientific) for RBD and Strep-Tactin®XT 4Flow® high capacity resin (IBA) for ACE2 ectodomains. The protein concentration was quantified using the BCA protein determination kit (EpiZyme) and
SDS-PAGE with Coomassie blue staining was employed for analysis. LIVE CELL RBD BINDING ASSAY HEK293T cells stably expressing ACE2 were seeded in poly-D-lysine-treated 96-well plates. After
12 hours, with cells were incubated with RBD-hFc protein (2 μg/mL) in growth medium for 0.5 hours at 4 °C. Subsequently cells were washed with Hanks’ Balanced Salt Solution (with Ca2+ &
Mg2 + )(HBSS) twice, and then treated with Alexa Fluor 488-conjugated goat anti-human IgG (catalogue no. A11013; Thermo Fisher Scientific) at a concentration of 2 μg/mL in DMEM with 2% FBS
for 30 minutes (min) at 4 °C. Hoechst 33342 (1:5,000 dilution in PBS) was utilized for nuclear staining. Following fixation with methanol at 25 °C for 5 minutes and washed once by HBSS,
images were captured by fluorescence microscopy (MI52-N; Mshot), and the fluorescence intensity was analyzed using Thermo Varioskan LUX Alexa. RFUs of each sample were normalized by
subjecting background signals in control cells (expressing hAPN) before analysis. The RFUs showing negative values after subtraction were presented as zero. FLOW CYTOMETRY HEK293T cells
stably expressing ACE2 orthologues (_R.aff, R.sha, R.alc, R.mal_, and _R.cor_) were cultured in 6-well plates for 12 hours. Cells were detached by 5 mM EDTA and washed twice by PBS, and then
incubated with indicated proteins (RaTG13 RBD, RshSTT200 RBD, BM48-31 WT RBD, BM48-31 A480Y RBD, RmYN05 RBD, Rc-o319 RBD with hFc tags) at a concentration of 20 μg/mL for 30 min at 4 °C.
Following three PBS washes, cells were stained with 488-conjugated goat anti-human IgG (1:1000, Alexa Fluor) for 30 min. Subsequently, flow cytometry analysis was performed using a CytoFLEX
analyzer, collecting 10,000 events per sample. In a separate assay demonstrating the sensitivity of live cell binding assay, HEK293T cells expressing hACE2 were plated 12 hours before
incubation with two-fold serial diluted SARS-CoV−2 RBD-hFc (from 20 μg/mL) for 30 min. After three PBS washes, cells were stained with 488-conjugated goat anti-human IgG (1:1000, Alexa
Fluor) and subjected to Flow cytometry analysis. For the pseudoviruses entry assays, GFP expressing VSV pseudotypes was 10-fold serial diluted from 1 × 106 TCID50/mL. After 12 hours
post-infection incubation, cells were washed with PBS and trypsinized for analysis. FlowJo V10 software was employed for data analysis. Gating strategy is illustrated in Supplementary Fig.
13. BIOLAYER INTERFEROMETRY (BLI) The Octet RED96 system (ForteBio, Menlo Park, CA) was employed to determine the apparent affinity (KD, app, due to the potential dimerization of ACE2)
between the RBD and ACE2. The buffer for analysis was phosphate buffer saline with 0.05% Tween20 (PBST). The RBD (10 μg/mL) was captured on ProA biosensors, followed by binding of ACE2
ectodomains at 2-fold serial dilutions ranging from 500 nM for 120 s, followed by dissociated in the PBST for additional 300 s. Analysis was conducted with curve-fitting kinetic with global
fitting with a 1:1 binding mode using ForteBio Octet analysis software v12.2.0.20 (ForteBio, Menlo Park, CA). Mean KD, app values were derived by averaging all binding curves that conformed
to the theoretical fit with an R2 value ≥ 0.95. PSEUDOVIRUS PRODUCTION AND ENTRY ASSAYS Pseudovirus incorporating coronaviruses S glycoproteins were produced using a vesicular stomatitis
virus (VSV)-based system with slight modifications to a well-established protocol73,76,77. In general, HEK293T cells were transfected with plasmids expressing S proteins through
Lipofectamine 2000 (Biosharp, China). After 24 hours, the transfected cells were infected with VSV-ΔG-fLuc-GFP (1×106 TCID50/mL) diluted in DMEM followed by a two-hour incubation on a shaker
at 37 °C, the cells were replenished with DMEM containing anti-VSV-G monoclonal antibody (I1, 1 μg/mL). After 24 hours, the pseudovirus-containing supernatant was harvested, centrifuged at
13,523 × g for 5 min at 4 °C, and stored at −80 °C. For the viral entry assay, the HEK293T cell lines expressing various ACE2 orthologues were inoculated with pseudotyped viruses in DMEM
with 10% FBS. In general, 3 × 104 trypsinized cells were incubated with pseudovirus (1.5 × 105 TCID50/100 μL) in a 96-well plate to allow cell attachment and pseudovirus entry. At 16-20 hpi
(hours post infection), images of the infected cells were captured by a fluorescence microscope (MI52-N; Mshot). Intracellular luciferase activity was determined using a Bright-Glo
Luciferase Assay Kit (Promega Corporation, E2620) and measured with a Thermo Varioskan LUX, SpectraMax iD3 Multi-well Luminometer (Molecular Devices) or a GloMax 20/20 Luminometer (Promega
Corporation). PCVSV-COV AMPLIFICATION ASSAY Plasmids for rescuing propagation-competent (pc) VSV-CoV genetically encoding HKU3 and HKU3 S + NNSVGD + RBM spike glycoproteins were generated by
replacing the fLuc coding sequences in the vector pVSV-ΔG-fLuc-GFP with coronavirus spike coding sequences75. Reverse genetics experiments were conducted to rescue recombinant pcVSV-CoV
according to a previously described protocol77. BHK-21 cells of 80% confluence in a 6-well plate were infected with recombinant vaccinia virus expressing T7 RNA polymerase (VVT7, a gift from
Mingzhou Chen’s lab, Wuhan University) at a multiplicity of infection (MOI) of 5 for 45 minutes at 37 °C. Subsequently, the vvT7 was removed by PBS wash, and the cells were transfected with
the pVSV-dG-GFP-S plasmids and helper plasmids in a ratio of 5:3:5:8:1 (pVSV-dG-GFP-S: pBS-N: pBS-P: pBS-G: pBS-L). Supernatant containing pcVSV-CoV (P0) was collected at 48 hours
post-transfection and filtered through a 0.22-μm filter to remove vvT7. Subsequently, Caco2 cells transfected with plasmids expressing VSV-G for 24 hours were further infected with P0
supernatant for VSV-G efficient virus amplification assisted by VSV-G, which generated the passage 1 (P1) supernatant. P1 viruses were further amplified in Caco2 cells stably expressing
either hACE2 or an HKU3-customized viral receptor, in the presence of anti-VSV-G antibody (I1-Hybridoma supernatant), resulting in passage 2 (P2) viruses carrying HKU3 S + NNSVGD +
RBMSARS-CoV-2 or HKU3 S glycoproteins, respectively78. For a typical virus amplification assay, 3 × 104 trypsinized cells were incubated with pcVSV-CoV (1 × 104 TCID50/100 μL) in a 96-well
plate. For the hACE2 antibody h11B11 neutralization assay, cells were incubated with indicated concentrations of antibody for 1 hour at 4 °C, washed by PBS, and then incubated with viruses.
GFP images were captured after 24 hours post-infection. AUTHENTIC VIRUS INFECTION The SARS-CoV-2 WT strain (IVCAS 6.7512) was provided by the National Virus Resource, Wuhan Institute of
Virology, Chinese Academy of Sciences. The BA.1 strain (YJ20220223) was provided by Hubei Provincial Center for Disease Control and Prevention. SARS-CoV-2 authentic viruses-related
experiments were conducted in ABSL-3 facility at Wuhan University with the approval from the Biosafety Committee of ABSL-3 lab. HEK293T cells expressing ACE2 orthologues were seeded in
poly-lysine-treated 96-well plates (1.25 × 105 cells/well). At 12 hours post seeding, SARS-CoV-2 strains (WT and Omicron BA.1) were introduced to different stable cells and incubated for 1-2
hours. Following a medium change to DMEM with 2% FBS, cells were cultured for 24 hours, fixed with methanol, and treated with anti-SARS-CoV-2 Nucleocapsid (N) antibody (catalogue no.
40143-MM05; Sino Biological) at 1:1000 for one hour at 37 °C. After PBS wash, cells were treated with a secondary antibody (Alexa Fluor 594) and Hoechst 33342 (1:10,000 dilution in PBS) for
nuclei staining. Images were captured using a fluorescence microscope (MI52-N, Mshot, China). STRUCTURAL ANALYSIS Protein structures were predicted by AlphaFold2 and HDOCK79,80,81. Briefly,
AlphaFold2, implemented in ColabFold, was utilized with default settings for predicting the protein structures of various sarbecovirus RBDs and ACE2 orthologues. The top-ranked model was
used for all subsequent analyses. The docking of the ACE2 ectodomain in complex with RBD was accomplished using HDOCK (v.1.1). Structural representations and analyses were carried out within
ChimeraX (v.1.8). The hydrogen bonds and clashes between the displayed amino acids were analyzed using the H-bonds and clashes command. The following cryo-EM complex structures in the PDB
database were also used for structural analysis in this study: human ACE2/SARS-CoV-2-RBD (Protein Data Bank 6M0J), human ACE2/SARS-CoV-1-RBD (3SCI), human ACE2/RaTG13-RBD (7DRV), human
ACE2/GX_P2V-RBD (7DDP), human ACE2/SARS-CoV-2 alpha variant-RBD (7EKF), human ACE2/RshSTT200-RBD (7XBH), Rhinolophus alcyone ACE2/PRD-0038-RBD (8U0T), and RsYN04 RBD/antibody S43 (8J5J).
WESTERN BLOT To examine the intracellular sarbecoviruses S glycoprotein expression levels, HEK293T cells were transfected with plasmids encoding the viral S glycoproteins fused with a
C-terminal HA-tag. After 24 hours, cells were washed with PBS, lysed on ice for 10 min in 2% TritonX-100/PBS containing 1 mM PMSF (Beyotime, ST506). The cell lysates were clarified by
centrifugation at 13,523 × g for 5 mins at 4 °C. The supernatants were mixed with 1:5 (v/v) 5× SDS-loading buffer and incubated at 95 °C for 5 min. For evaluating the S glycoprotein levels
in pseudovirus (PSV) particles in the cultured medium, PSV was concentrated with a 30% sucrose cushion (30% sucrose, 15 mM Tris–HCl, 100 mM NaCl, 0.5 mM EDTA) at 20,000 x g for 1.5 hours at
4 °C. The concentrated PSV was then resuspended in 1×SDS loading buffer and incubated at 95 °C for 30 min. Following SDS-PAGE and PVDF membrane transfer, the blots were blocked with 10% milk
in TBST containing 0.1% TBS (20 mM Tris-HCl pH 8.0, 150 mM NaCl) supplemented with 0.05% Tween-20 at room temperature for 1 hour. Primary antibodies targeting HA (MBL, MBL-M180-3),
β-tubulin (Immunoway, YM3030), or VSV-M (Kerafast, EB0011) were applied at a 1:10,000 dilution in TBST with 1% milk. After three washes with TBST, blots were incubated with the secondary
antibody Peroxidase AffiniPure Goat Anti-Mouse IgG (H + L) (Jackson Immuno Research, 115-035-003). Blots were further washed three times before chemiluminescence detection (SQ201, Yamei
Biotech) using the ChemiDoc MP Imaging System (Bio-Rad). Uncropped scans of all blots in the Figures are supplied in the Source Data file, and the uncropped scans of those presented in the
Supplementary Figs. were included at the end of the Supplementary Information file (Supplementary Fig. 14). GEOGRAPHICAL DISTRIBUTION OF BAT SPECIES The global distribution data of bat
species were obtained from the IUCN Red List of Threatened Species 2020, the base layer of the map (version 5.1.1) was sourced from Natural Earth, available at
(https://www.naturalearthdata.com/downloads/110mcultural-vectors/). GeoScene Pro 21 was utilized to visualize and analyze the bat distribution data. STATISTICAL AND REPRODUCIBILITY Most
experiments were independently conducted 2−4 times with similar results, each with 2-3 biologically independent replicates. Representative results were shown. Heat maps were plotted based on
combined data of mean values (at least _n_ = 2 biologically independent wells of cells) of RLU or RFU that were obtained in one or several experiments, with background (control cells
expressing APN) signals subtracted. Most data are presented as means ± standard deviation (s.d.) as indicated in the figure legends. All statistical analyses were conducted using Prism 9
software (GraphPad). Two-tailed unpaired (Student’s) _t_-test was performed if only two conditions were compared. One-way ANOVA analysis, followed by Dunnett’s test, was employed for
multiple comparisons. The association between the entry/binding efficiency and the presence of RBM disulfide was assessed using the two-sided chi-squared test. _P_ < 0.05 was considered
significant. *_P_ < 0.05, **_P_ < 0.01, ***_P_ < 0.005, and ****_P_ < 0.001; NS, not significant (_P_ > 0.05). REPORTING SUMMARY Further information on research design is
available in the Nature Portfolio Reporting Summary linked to this article. DATA AVAILABILITY The authors declare that the data supporting the findings of this study are available within the
paper and its supplementary information files. Bat distribution shapefiles are available at https://www.iucnredlist.org/search?query=bat&searchType=species. The structural models of the
ACE2 protein and RBD protein were downloaded from Protein Data Bank with PDB ID: human ACE2/SARS-CoV-2-RBD (6M0J), human ACE2/SARS-CoV-1-RBD (3SCI), human ACE2/RaTG13-RBD (7DRV), human
ACE2/GX_P2V-RBD (7DDP), human ACE2/SARS-CoV-2 alpha variant-RBD (7EKF), human ACE2/RshSTT200-RBD (7XBH), Rhinolophus alcyone ACE2/PRD-0038-RBD (8U0T), and RsYN04 RBD/antibody S43 (8J5J). The
accession numbers of 268 sarbecovirus spike proteins and 56 ACE2 orthologues used in this study are available in the Supplementary Data 1. The data generated in this study are provided in
the Supplementary Information. Uncropped scans of all blots in the Supplementary Figs. were included at the end of the Supplementary Information file (Supplementary Fig. 14). Source data are
provided with this paper. REFERENCES * Ksiazek, T. G. et al. A novel coronavirus associated with severe acute respiratory syndrome. _N. Engl. J. Med._ 348, 1953–1966 (2003). Article PubMed
CAS Google Scholar * Zhou, P. et al. A pneumonia outbreak associated with a new coronavirus of probable bat origin. _Nature_ 579, 270–273 (2020). Article ADS PubMed CAS Google
Scholar * Starr, T. N. et al. ACE2 binding is an ancestral and evolvable trait of sarbecoviruses. _Nature_ 603, 913–918 (2022). Article ADS PubMed PubMed Central CAS Google Scholar *
Letko, M., Marzi, A. & Munster, V. Functional assessment of cell entry and receptor usage for SARS-CoV-2 and other lineage B betacoronaviruses. _Nat. Microbiol_. 5, 562–569 (2020).
Article PubMed PubMed Central CAS Google Scholar * Murakami, S. et al. Isolation of Bat Sarbecoviruses, Japan. _Emerg. Infect. Dis._ 28, 2500–2503 (2022). Article PubMed PubMed
Central CAS Google Scholar * Guo, H. et al. Identification of a novel lineage bat SARS-related coronaviruses that use bat ACE2 receptor. _Emerg. Microbes Infect._ 10, 1507–1514 (2021).
Article PubMed PubMed Central CAS Google Scholar * Zhou, H. et al. Identification of novel bat coronaviruses sheds light on the evolutionary origins of SARS-CoV-2 and related viruses.
_Cell_ 184, 4380–4391.e14 (2021). Article PubMed PubMed Central CAS Google Scholar * Wacharapluesadee, S. et al. Evidence for SARS-CoV-2 related coronaviruses circulating in bats and
pangolins in Southeast Asia. _Nat. Commun._ 12, 972 (2021). Article ADS PubMed PubMed Central CAS Google Scholar * Alkhovsky, S. et al. SARS-like Coronaviruses in Horseshoe Bats
(Rhinolophus spp.) in Russia, 2020. _Viruses_ 14, 113 (2022). * Lam, T. T.-Y. et al. Identifying SARS-CoV-2-related coronaviruses in Malayan pangolins. _Nature_ 583, 282–285 (2020). Article
ADS PubMed CAS Google Scholar * Meta Djomsi, D. et al. Coronaviruses Are Abundant and Genetically Diverse in West and Central African Bats, including Viruses Closely Related to Human
Coronaviruses. _Viruses_ 15, 337 (2023). * Murakami, S. et al. Detection and Characterization of Bat Sarbecovirus Phylogenetically Related to SARS-CoV-2, Japan. _Emerg. Infect. Dis._ 26,
3025–3029 (2020). Article PubMed CAS Google Scholar * Temmam, S. et al. Bat coronaviruses related to SARS-CoV-2 and infectious for human cells. _Nature_ 604, 330–336 (2022). Article ADS
PubMed CAS Google Scholar * Drexler, J. F. et al. Genomic characterization of severe acute respiratory syndrome-related coronavirus in European bats and classification of coronaviruses
based on partial RNA-dependent RNA polymerase gene sequences. _J. Virol._ 84, 11336–11349 (2010). Article PubMed CAS Google Scholar * Hu, B. et al. Discovery of a rich gene pool of bat
SARS-related coronaviruses provides new insights into the origin of SARS coronavirus. _PLoS Pathog_ 13, e1006698 (2017). Article PubMed PubMed Central Google Scholar * Ge, X.-Y. et al.
Isolation and characterization of a bat SARS-like coronavirus that uses the ACE2 receptor. _Nature_ 503, 535–538 (2013). Article ADS PubMed PubMed Central CAS Google Scholar * Li, W.
et al. Bats are natural reservoirs of SARS-like coronaviruses. _Science_ 310, 676–679 (2005). Article ADS PubMed CAS Google Scholar * Xiao, K. et al. Isolation of SARS-CoV-2-related
coronavirus from Malayan pangolins. _Nature_ 583, 286–289 (2020). Article ADS PubMed CAS Google Scholar * Guo, Z. et al. SARS-CoV-2-related pangolin coronavirus exhibits similar
infection characteristics to SARS-CoV-2 and direct contact transmissibility in hamsters. _iScience_ 25, 104350 (2022). Article ADS PubMed PubMed Central CAS Google Scholar * Niu, S. et
al. Molecular basis of cross-species ACE2 interactions with SARS-CoV-2-like viruses of pangolin origin. _EMBO J_ 40, e107786 (2021). Article PubMed PubMed Central CAS Google Scholar *
Li, P. et al. The Rhinolophus affinis bat ACE2 and multiple animal orthologs are functional receptors for bat coronavirus RaTG13 and SARS-CoV-2. _Sci Bull (Beijing)_ 66, 1215–1227 (2021).
Article ADS PubMed CAS Google Scholar * Liu, K. et al. Binding and molecular basis of the bat coronavirus RaTG13 virus to ACE2 in humans and other species. _Cell_ 184, 3438–3451.e10
(2021). Article PubMed PubMed Central CAS Google Scholar * Boni, M. F. et al. Evolutionary origins of the SARS-CoV-2 sarbecovirus lineage responsible for the COVID-19 pandemic. _Nat.
Microbiol_. 5, 1408–1417 (2020). Article PubMed CAS Google Scholar * Hofmann, H. et al. Human coronavirus NL63 employs the severe acute respiratory syndrome coronavirus receptor for
cellular entry. _Proc. Natl. Acad. Sci. USA._ 102, 7988–7993 (2005). Article ADS PubMed PubMed Central CAS Google Scholar * Xiong, Q. et al. Close relatives of MERS-CoV in bats use
ACE2 as their functional receptors. _Nature_ 612, 748–757 (2022). Article ADS PubMed PubMed Central CAS Google Scholar * Tan, C. C. S. et al. Genomic screening of 16 UK native bat
species through conservationist networks uncovers coronaviruses with zoonotic potential. _Nat. Commun._ 14, 3322 (2023). Article ADS PubMed PubMed Central CAS Google Scholar * Hu, Y.
et al. Host range and structural analysis of bat-origin RshSTT182/200 coronavirus binding to human ACE2 and its animal orthologs. _EMBO J_ 42, e111737 (2023). Article PubMed PubMed Central
CAS Google Scholar * Lee, J. et al. Broad receptor tropism and immunogenicity of a clade 3 sarbecovirus. _Cell Host Microbe_ 31, 1961–1973.e11 (2023). Article PubMed CAS Google
Scholar * Lan, J. et al. Structure of the SARS-CoV-2 spike receptor-binding domain bound to the ACE2 receptor. _Nature_ 581, 215–220 (2020). Article ADS PubMed CAS Google Scholar * Wu,
K., Peng, G., Wilken, M., Geraghty, R. J. & Li, F. Mechanisms of host receptor adaptation by severe acute respiratory syndrome coronavirus. _J. Biol. Chem._ 287, 8904–8911 (2012).
Article PubMed PubMed Central CAS Google Scholar * Lu, G., Wang, Q. & Gao, G. F. Bat-to-human: spike features determining ‘host jump’ of coronaviruses SARS-CoV, MERS-CoV, and
beyond. _Trends Microbiol_. 23, 468–478 (2015). Article PubMed PubMed Central CAS Google Scholar * Wu, Z. et al. A comprehensive survey of bat sarbecoviruses across China in relation to
the origins of SARS-CoV and SARS-CoV-2. _Natl Sci. Rev_. 10, nwac213 (2023). Article PubMed CAS Google Scholar * Yan, H. et al. ACE2 receptor usage reveals variation in susceptibility
to SARS-CoV and SARS-CoV-2 infection among bat species. _Nat Ecol Evol_ 5, 600–608 (2021). Article PubMed Google Scholar * Liu, Y. et al. Functional and genetic analysis of viral receptor
ACE2 orthologs reveals a broad potential host range of SARS-CoV-2. _Proc. Natl. Acad. Sci. USA_. 118, e2025373118 (2021). * Zhang, Y. et al. Cross-species tropism and antigenic landscapes
of circulating SARS-CoV-2 variants. _Cell Rep_. 38, 110558 (2022). Article PubMed PubMed Central CAS Google Scholar * Latinne, A. et al. Origin and cross-species transmission of bat
coronaviruses in China. _Nat. Commun._ 11, 4235 (2020). Article ADS PubMed PubMed Central CAS Google Scholar * Wu, L. et al. Broad host range of SARS-CoV-2 and the molecular basis for
SARS-CoV-2 binding to cat ACE2. _Cell Discov_. 6, 68 (2020). Article PubMed PubMed Central CAS Google Scholar * Li, Y. et al. SARS-CoV-2 and Three Related Coronaviruses Utilize Multiple
ACE2 Orthologs and Are Potently Blocked by an Improved ACE2-Ig. _J. Virol_. 94, e01283–20 (2020). * Li, S. et al. Cross-species recognition and molecular basis of SARS-CoV-2 and SARS-CoV
binding to ACE2s of marine animals. _Natl Sci. Rev_. 9, nwac122 (2022). Article PubMed PubMed Central CAS Google Scholar * Mou, H. et al. Mutations derived from horseshoe bat ACE2
orthologs enhance ACE2-Fc neutralization of SARS-CoV-2. _PLoS Pathog_ 17, e1009501 (2021). Article PubMed PubMed Central CAS Google Scholar * Conceicao, C. et al. The SARS-CoV-2 Spike
protein has a broad tropism for mammalian ACE2 proteins. _PLoS Biol_. 18, e3001016 (2020). Article PubMed PubMed Central CAS Google Scholar * Chen, J. et al. The binding and structural
basis of fox ACE2 to RBDs from different sarbecoviruses. _Virol. Sin_. https://doi.org/10.1016/j.virs.2024.06.004 (2024). * Li, P. et al. Effect of polymorphism in Rhinolophus affinis ACE2
on entry of SARS-CoV-2 related bat coronaviruses. _PLoS Pathog_ 19, e1011116 (2023). Article PubMed PubMed Central CAS Google Scholar * Guo, H. et al. Evolutionary Arms Race between
Virus and Host Drives Genetic Diversity in Bat Severe Acute Respiratory Syndrome-Related Coronavirus Spike Genes. _J. Virol_. 94, e00902–20 (2020). * Zech, F. et al. Spike residue 403
affects binding of coronavirus spikes to human ACE2. _Nat. Commun._ 12, 6855 (2021). Article ADS PubMed PubMed Central CAS Google Scholar * Wang, Q. et al. Determinants of
species-specific utilization of ACE2 by human and animal coronaviruses. _Commun Biol_. 6, 1051 (2023). Article PubMed PubMed Central CAS Google Scholar * Kang, L. et al. A selective
sweep in the Spike gene has driven SARS-CoV-2 human adaptation. _Cell_ 184, 4392–4400.e4 (2021). Article PubMed PubMed Central CAS Google Scholar * Ou, X. et al. Host susceptibility and
structural and immunological insight of S proteins of two SARS-CoV-2 closely related bat coronaviruses. _Cell Discov_. 9, 78 (2023). Article PubMed PubMed Central CAS Google Scholar *
Delaune, D. et al. A novel SARS-CoV-2 related coronavirus in bats from Cambodia. _Nat. Commun._ 12, 6563 (2021). Article ADS PubMed PubMed Central CAS Google Scholar * Guo, H. et al.
ACE2-Independent Bat Sarbecovirus Entry and Replication in Human and Bat Cells. _MBio_ 13, e0256622 (2022). Article PubMed Google Scholar * Roelle, S. M., Shukla, N., Pham, A. T.,
Bruchez, A. M. & Matreyek, K. A. Expanded ACE2 dependencies of diverse SARS-like coronavirus receptor binding domains. _PLoS Biol_. 20, e3001738 (2022). Article PubMed PubMed Central
CAS Google Scholar * Hu, D. et al. Genomic characterization and infectivity of a novel SARS-like coronavirus in Chinese bats. _Emerg. Microbes Infect._ 7, 154 (2018). Article PubMed
PubMed Central Google Scholar * Ren, W. et al. Difference in receptor usage between severe acute respiratory syndrome (SARS) coronavirus and SARS-like coronavirus of bat origin. _J.
Virol._ 82, 1899–1907 (2008). Article PubMed CAS Google Scholar * Wells, H. L. et al. The evolutionary history of ACE2 usage within the coronavirus subgenus Sarbecovirus. _Virus Evol_ 7,
veab007 (2021). Article PubMed PubMed Central CAS Google Scholar * Crook, J. M. et al. Metagenomic identification of a new sarbecovirus from horseshoe bats in Europe. _Sci. Rep._ 11,
14723 (2021). Article ADS PubMed CAS Google Scholar * Tao, Y. & Tong, S. Complete Genome Sequence of a Severe Acute Respiratory Syndrome-Related Coronavirus from Kenyan Bats.
_Microbiol Resour Announc_ 8, e00548–19 (2019). * Su, C. et al. Structural characteristics of BtKY72 RBD bound to bat ACE2 reveal multiple key residues affecting ACE2 usage of
sarbecoviruses. _MBio_15, e0140424 (2024). * Seifert, S. N. et al. An ACE2-dependent Sarbecovirus in Russian bats is resistant to SARS-CoV-2 vaccines. _PLoS Pathog_ 18, e1010828 (2022).
Article PubMed CAS Google Scholar * Grishin, A. M. et al. Disulfide Bonds Play a Critical Role in the Structure and Function of the Receptor-binding Domain of the SARS-CoV-2 Spike
Antigen. _J. Mol. Biol._ 434, 167357 (2022). Article PubMed CAS Google Scholar * Zhang, S. et al. Loss of Spike N370 glycosylation as an important evolutionary event for the enhanced
infectivity of SARS-CoV-2. _Cell Res_. 32, 315–318 (2022). Article PubMed PubMed Central CAS Google Scholar * Wang, Q. et al. Key mutations on spike protein altering ACE2 receptor
utilization and potentially expanding host range of emerging SARS-CoV-2 variants. _J. Med. Virol._ 95, e28116 (2023). Article PubMed CAS Google Scholar * Zhao, Z. et al. Structural basis
for receptor binding and broader interspecies receptor recognition of currently circulating Omicron sub-variants. _Nat. Commun._ 14, 4405 (2023). Article ADS PubMed PubMed Central CAS
Google Scholar * Starr, T. N. et al. Shifting mutational constraints in the SARS-CoV-2 receptor-binding domain during viral evolution. _Science_ 377, 420–424 (2022). Article ADS PubMed
CAS Google Scholar * Han, P. et al. Molecular insights into receptor binding of recent emerging SARS-CoV-2 variants. _Nat. Commun._ 12, 6103 (2021). Article ADS PubMed PubMed Central
CAS Google Scholar * Hati, S. & Bhattacharyya, S. Impact of Thiol-Disulfide Balance on the Binding of Covid-19 Spike Protein with Angiotensin-Converting Enzyme 2 Receptor. _ACS Omega_
5, 16292–16298 (2020). Article PubMed PubMed Central CAS Google Scholar * Gao, B. & Zhu, S. Mutation-driven parallel evolution in emergence of ACE2-utilizing sarbecoviruses. _Front.
Microbiol._ 14, 1118025 (2023). Article PubMed PubMed Central Google Scholar * Du, Y. et al. A broadly neutralizing humanized ACE2-targeting antibody against SARS-CoV-2 variants. _Nat.
Commun._ 12, 5000 (2021). Article ADS PubMed PubMed Central CAS Google Scholar * Mykytyn, A. Z., Fouchier, R. A. & Haagmans, B. L. Antigenic evolution of SARS coronavirus 2. _Curr.
Opin. Virol._ 62, 101349 (2023). Article PubMed CAS Google Scholar * Menachery, V. D. et al. Trypsin Treatment Unlocks Barrier for Zoonotic Bat Coronavirus Infection. _J. Virol_. 94,
e01774–19 (2020). * Richard, M. et al. Factors determining human-to-human transmissibility of zoonotic pathogens via contact. _Curr. Opin. Virol._ 22, 7–12 (2017). Article PubMed Google
Scholar * Nakamura, T., Yamada, K. D., Tomii, K. & Katoh, K. Parallelization of MAFFT for large-scale multiple sequence alignments. _Bioinformatics_ 34, 2490–2492 (2018). Article
PubMed PubMed Central CAS Google Scholar * Nguyen, L.-T., Schmidt, H. A., von Haeseler, A. & Minh, B. Q. IQ-TREE: a fast and effective stochastic algorithm for estimating
maximum-likelihood phylogenies. _Mol. Biol. Evol._ 32, 268–274 (2015). Article PubMed CAS Google Scholar * Schwegmann-Weßels, C. et al. Comparison of vesicular stomatitis virus
pseudotyped with the S proteins from a porcine and a human coronavirus. _J. Gen. Virol._ 90, 1724–1729 (2009). Article PubMed Google Scholar * Fukushi, S. et al. Vesicular stomatitis
virus pseudotyped with severe acute respiratory syndrome coronavirus spike protein. _J. Gen. Virol._ 86, 2269–2274 (2005). Article PubMed CAS Google Scholar * Ma, C. et al. Broad host
tropism of ACE2-using MERS-related coronaviruses and determinants restricting viral recognition. _Cell Discov_. 9, 57 (2023). Article PubMed PubMed Central Google Scholar * Nie, J. et
al. Quantification of SARS-CoV-2 neutralizing antibody by a pseudotyped virus-based assay. _Nat. Protoc._ 15, 3699–3715 (2020). Article PubMed CAS Google Scholar * Whitt, M. A.
Generation of VSV pseudotypes using recombinant ΔG-VSV for studies on virus entry, identification of entry inhibitors, and immune responses to vaccines. _J. Virol. Methods_ 169, 365–374
(2010). Article PubMed PubMed Central CAS Google Scholar * Liu, P. et al. Engineering customized viral receptors for various coronaviruses. _bioRxiv_ 2024.03.03.583237 (2024)
https://doi.org/10.1101/2024.03.03.583237. * Jumper, J. et al. Highly accurate protein structure prediction with AlphaFold. _Nature_ 596, 583–589 (2021). Article ADS PubMed PubMed Central
CAS Google Scholar * Yan, Y., Tao, H., He, J. & Huang, S.-Y. The HDOCK server for integrated protein-protein docking. _Nat. Protoc._ 15, 1829–1852 (2020). Article PubMed CAS
Google Scholar * Yan, Y., Wen, Z., Wang, X. & Huang, S.-Y. Addressing recent docking challenges: A hybrid strategy to integrate template-based and free protein-protein docking.
_Proteins_ 85, 497–512 (2017). Article PubMed CAS Google Scholar Download references ACKNOWLEDGEMENTS We are grateful to the funding support from National Key R&D Program of China
(2023YFC2605500 to H.Y.), National Natural Science Foundation of China (NSFC) projects (82322041, 32270164, 32070160 to H.Y. and 323B2006 to C.B.M), Fundamental Research Funds for the
Central Universities (2042023kf0191, 2042022kf1188, and 2042024kf1028 to H.Y.), Natural Science Foundation of Hubei Province (2023AFA015 to H.Y.), Hubei Provincial Research Center of Basic
Discipline of Biology (to H.Y.) and TaiKang Center for Life and Medical Sciences (to H.Y.). We thank Huabin Zhao (Wuhan University) for his help in providing the coding sequences of many bat
ACE2 orthologues. We thank Ming Guo (Wuhan University) for his help in conducting SARS-CoV-2 authentic viruses-related experiments in ABSL-3. We thank Qiang Ding (Tsinghua University) for
his kind offer of several plasmids expressing mammalian ACE2 orthologues. We also want to express our gratitude to the core facilities and ABSL-3 laboratory of the Key Laboratory of
Virology, Wuhan University. AUTHOR INFORMATION Author notes * These authors contributed equally: Jun-Yu Si, Yuan-Mei Chen, Ye-Hui Sun. AUTHORS AND AFFILIATIONS * State Key Laboratory of
Virology, College of Life Sciences, TaiKang Center for Life and Medical Sciences, Wuhan University, Wuhan, Hubei, China Jun-Yu Si, Yuan-Mei Chen, Ye-Hui Sun, Meng-Xue Gu, Mei-Ling Huang,
Lu-Lu Shi, Xiao Yu, Xiao Yang, Qing Xiong, Cheng-Bao Ma, Peng Liu & Huan Yan * Wuhan Institute of Virology, Chinese Academy of Sciences, Wuhan, China Zheng-Li Shi * Guangzhou Laboratory,
Guangzhou International Bio Island, Guangzhou, China Zheng-Li Shi Authors * Jun-Yu Si View author publications You can also search for this author inPubMed Google Scholar * Yuan-Mei Chen
View author publications You can also search for this author inPubMed Google Scholar * Ye-Hui Sun View author publications You can also search for this author inPubMed Google Scholar *
Meng-Xue Gu View author publications You can also search for this author inPubMed Google Scholar * Mei-Ling Huang View author publications You can also search for this author inPubMed Google
Scholar * Lu-Lu Shi View author publications You can also search for this author inPubMed Google Scholar * Xiao Yu View author publications You can also search for this author inPubMed
Google Scholar * Xiao Yang View author publications You can also search for this author inPubMed Google Scholar * Qing Xiong View author publications You can also search for this author
inPubMed Google Scholar * Cheng-Bao Ma View author publications You can also search for this author inPubMed Google Scholar * Peng Liu View author publications You can also search for this
author inPubMed Google Scholar * Zheng-Li Shi View author publications You can also search for this author inPubMed Google Scholar * Huan Yan View author publications You can also search for
this author inPubMed Google Scholar CONTRIBUTIONS Conceptualization, H.Y., and J.Y.S.; methodology, J.Y.S., Y.M.C, Y.H.S, M.X.G, C.L.W., C.B.M, P. L., Q.X., L.L.S., M.L.H., X.Yu., X.Yang.,
Z.X.M., Y.C.S.; data analysis, J.Y.S., Y.M.C., Y.H.S., M.X.G.; writing—original draft, H.Y., J.Y.S., Y.M.C.; writing—review & editing, H.Y., J.Y.S., Z.L.S.; supervision and funding
acquisition, H.Y. CORRESPONDING AUTHOR Correspondence to Huan Yan. ETHICS DECLARATIONS COMPETING INTERESTS The authors declare no competing interests. PEER REVIEW PEER REVIEW INFORMATION
_Nature Communications_ thanks Frank Kirchhoff and the other anonymous reviewer(s) for their contribution to the peer review of this work. A peer review file is available. ADDITIONAL
INFORMATION PUBLISHER’S NOTE Springer Nature remains neutral with regard to jurisdictional claims in published maps and institutional affiliations. SUPPLEMENTARY INFORMATION SUPPLEMENTARY
INFORMATION PEER REVIEW FILE REPORTING SUMMARY DESCRIPTION OF ADDITIONAL SUPPLEMENTARY FILES SUPPLEMENTARY DATA 1 SUPPLEMENTARY DATA 2 SOURCE DATA SOURCE DATA RIGHTS AND PERMISSIONS OPEN
ACCESS This article is licensed under a Creative Commons Attribution-NonCommercial-NoDerivatives 4.0 International License, which permits any non-commercial use, sharing, distribution and
reproduction in any medium or format, as long as you give appropriate credit to the original author(s) and the source, provide a link to the Creative Commons licence, and indicate if you
modified the licensed material. You do not have permission under this licence to share adapted material derived from this article or parts of it. The images or other third party material in
this article are included in the article’s Creative Commons licence, unless indicated otherwise in a credit line to the material. If material is not included in the article’s Creative
Commons licence and your intended use is not permitted by statutory regulation or exceeds the permitted use, you will need to obtain permission directly from the copyright holder. To view a
copy of this licence, visit http://creativecommons.org/licenses/by-nc-nd/4.0/. Reprints and permissions ABOUT THIS ARTICLE CITE THIS ARTICLE Si, JY., Chen, YM., Sun, YH. _et al._
Sarbecovirus RBD indels and specific residues dictating multi-species ACE2 adaptiveness. _Nat Commun_ 15, 8869 (2024). https://doi.org/10.1038/s41467-024-53029-3 Download citation *
Received: 06 March 2024 * Accepted: 24 September 2024 * Published: 14 October 2024 * DOI: https://doi.org/10.1038/s41467-024-53029-3 SHARE THIS ARTICLE Anyone you share the following link
with will be able to read this content: Get shareable link Sorry, a shareable link is not currently available for this article. Copy to clipboard Provided by the Springer Nature SharedIt
content-sharing initiative