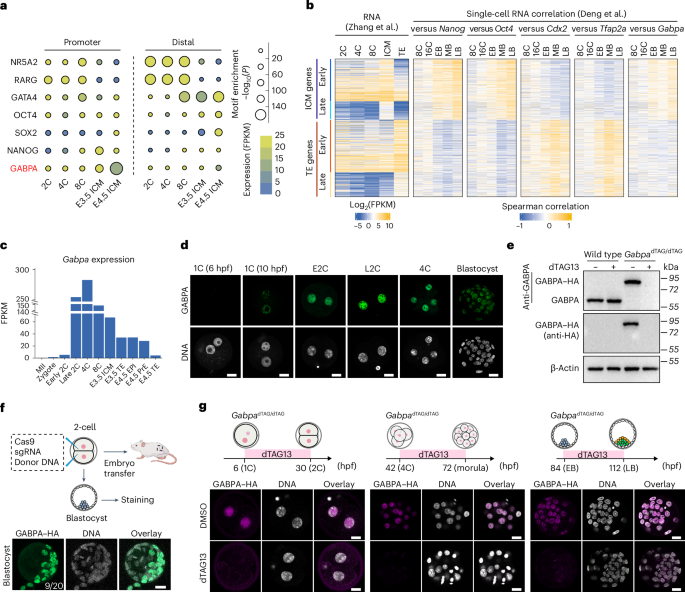
The transcription factor gabpa is a master regulator of naive pluripotency
- Select a language for the TTS:
- UK English Female
- UK English Male
- US English Female
- US English Male
- Australian Female
- Australian Male
- Language selected: (auto detect) - EN
Play all audios:

ABSTRACT The establishment of naive pluripotency is a continuous process starting with the generation of inner cell mass (ICM) that then differentiates into epiblast (EPI). Recent studies
have revealed key transcription factors (TFs) for ICM formation, but which TFs initiate EPI specification remains unknown. Here, using a targeted rapid protein degradation system, we show
that GABPA is not only a regulator of major ZGA, but also a master EPI specifier required for naive pluripotency establishment by regulating 47% of EPI genes during E3.5 to E4.5 transition.
Chromatin binding dynamics analysis suggests that GABPA controls EPI formation at least partly by binding to the ICM gene promoters occupied by the pluripotency regulators TFAP2C and SOX2 at
E3.5 to establish naive pluripotency at E4.5. Our study not only uncovers GABPA as a master pluripotency regulator, but also supports the notion that mammalian pluripotency establishment
requires a dynamic and stepwise multi-TF regulatory network. SIMILAR CONTENT BEING VIEWED BY OTHERS THE TRANSCRIPTION FACTOR OCT6 PROMOTES THE DISSOLUTION OF THE NAÏVE PLURIPOTENT STATE BY
REPRESSING _NANOG_ AND ACTIVATING A FORMATIVE STATE GENE REGULATORY NETWORK Article Open access 07 May 2024 _ZFP296_ KNOCKOUT ENHANCES CHROMATIN ACCESSIBILITY AND INDUCES A UNIQUE STATE OF
PLURIPOTENCY IN EMBRYONIC STEM CELLS Article Open access 24 July 2023 EXTENSIVE CO-BINDING AND RAPID REDISTRIBUTION OF NANOG AND GATA6 DURING EMERGENCE OF DIVERGENT LINEAGES Article Open
access 23 July 2022 MAIN The development of mouse embryos starts with maternal-to-zygotic transition, which is accompanied by maternal RNA degradation and zygotic genome activation (ZGA)1,2.
After a few rounds of cleavage, the totipotent embryos go through the first cell lineage differentiation to generate inner cell mass (ICM) and trophectoderm (TE) at embryonic day 3.5 (E3.5)
(refs. 3,4). Subsequently, the ICM cells go through the second cell lineage differentiation to generate epiblast (EPI) and primitive endoderm (PrE) at E4.5 (ref. 5). Transcription factors
(TFs) play important roles in cell lineage specification and pluripotency acquisition. At E3.5, the ICM cells express both pluripotency factors such as _Oct4_, _Sox2_ and _Nanog_ and PrE
factors such as _Gata4_ and _Gata6_6. After completion of the second cell fate specification at E4.5, the expression of lineage-specific TFs becomes restricted with _Gata4_ and _Gata6_
confined to the PrE, while _Nanog_ and _Sox2_ are restricted to the EPI, marking the establishment of naive pluripotency in EPI6. Given that embryonic stem (ES) cells cannot be fully
established from E3.5 ICM7, and E3.5 ICM cells have different transcriptome and chromatin accessibility from that of the E4.5 EPI8, E3.5 ICM is considered to be at a ‘pre-pluripotency’
state8. Recent studies indicate that the TFs NR5A2 and TFAP2C mediate totipotency to pluripotency transition by activating pre-pluripotency genes9. However, neither of them is responsible
for activating naive pluripotency genes10. Moreover, although NANOG and SOX2 are essential for maintaining the naive pluripotency state, they are not required for initiating the naive
pluripotency in vivo11,12. Thus, the TFs that drive ICM to naive pluripotency transition remain elusive. Identification of the TFs regulating pluripotency establishment in vivo is hindered
by their potential roles in earlier developmental stages. Thus, conventional TF-knockout (KO) mouse models could not separate their potential roles in regulating totipotency or ICM formation
from that in regulating EPI formation. The development of a targeted protein degradation system such as auxin-inducible degron (AID)13 and degradation tag (dTAG)14,15 enables the rapid
degradation of target proteins at a specific time window, making the study of gene function at a specific developmental stage possible. In this study, by generating dTAG mice and combining
with RNA sequencing (RNA-seq) and low-input CUT&RUN assay, we identified and demonstrated that the TF GA repeat binding protein alpha (GABPA), encoded by the minor ZGA gene _Gabpa_,
plays an essential role in naive pluripotency establishment in vivo. Our results revealed that GABPA is not only important for major ZGA, but also critical for ICM to naive pluripotency
transition by regulating a large set of pluripotency genes through binding to their promoters. Importantly, we found that, during ICM-to-EPI transition, the decreased binding of TFAP2C and
SOX2 at the promoters of certain ICM genes was concomitant with the increased binding of GABPA at these gene promoters, indicating a switch in the key TFs that regulate pluripotency gene
expression. These results support a dynamic and stepwise regulatory model for naive pluripotency establishment during pre-implantation development. RESULTS IDENTIFICATION OF GABPA AS A
POTENTIAL PLURIPOTENCY REGULATOR To identify candidate TFs potentially involved in ICM to naive EPI differentiation, we performed integrative analyses of public RNA-seq and assay for
transposase-accessible chromatin with sequencing (ATAC–seq) datasets from mouse pre-implantation embryos16,17,18,19. Compared with OCT4, NANOG and SOX2 binding motif, GABPA binding motif is
highly enriched in the open promoters of E4.5 ICM (EPI + PrE), suggesting GABPA may have a role in regulating E4.5 ICM formation (Fig. 1a). Consistent with a previous study16, GABPA binding
motif is not enriched in distal open chromatin (Fig. 1a). In addition, single-cell gene expression correlation analysis between various TFs and the ICM genes or TE genes (Supplementary Table
1) revealed that _Gabpa_ expression positively correlates with expression of ICM genes in blastocysts, particularly at late blastocyst stage similar to that of _Oct4_ and _Nanog_ (Fig. 1b),
indicating potential involvement of _Gabpa_ in pluripotency regulation. Furthermore, _Gabpa_ transcription starts from the zygote to the early two-cell stage, reaches its highest level at
late two-cell and four-cell stages, and then becomes differentially expressed in ICM and TE at the blastocyst stage (Fig. 1c). Immunostaining indicated that GABPA can be detected from zygote
to blastocyst (Fig. 1d). These data are consistent with a potential role of GABPA in regulating pluripotency. GABPA is a member of the erythroblast transformation specific (ETS) TF family,
which forms a tetrameric complex with GABPB to regulate the transcriptional activation20. Previous studies have shown that _Gabpa_ KO resulted in embryonic lethality before the blastocyst
stage21, but its role in cell fate specification is unknown. To assess its role in ICM and EPI specification, we utilized the dTAG system14. Given the potential instability of the dTAG
fusion protein22, we first tested the GABPA dTAG system in mouse ES cells, which showed that GABPA–FKBP (FK506 binding proteins)–HA (hemagglutinin) and the endogenous GABPA proteins are at
similar levels (Fig. 1e and Extended Data Fig. 1a), indicating that the FKBP–HA fusion does not affect GABPA stability. Importantly, dTAG13 treatment resulted in complete GABPA–FKBP–HA
degradation within 30 min (Fig. 1e). We thus proceeded to generate the mouse harbouring the _Gabpa_dTAG allele using the CRISPR technology (Fig. 1f and Extended Data Fig. 1a,b)23.
Importantly, addition of dTAG13 to the cultured embryos can efficiently degrade GABPA at zygote, two-cell, four-cell, morula and blastocyst stages in both short- and long-time-window
treatments (Fig. 1g and Extended Data Fig. 1c,d). Moreover, dTAG13 treatment did not affect the development of wide-type embryos (Extended Data Fig. 1e). Collectively, these results
demonstrate the successful generation of a GABPA-dTAG mouse model. GABPA ACTIVATES A GROUP OF MAJOR ZGA GENES BY BINDING TO THEIR PROMOTERS To understand when and how GABPA degradation
affects embryonic development, we performed dTAG13 treatment at different time windows. Given that _Gabpa_ starts to express after fertilization (Fig. 1c), we confirmed that GABPA is a minor
ZGA gene with a detectable protein level at 10 h post fertilization (hpf) (Fig. 1d). We therefore performed GABPA degradation starting at 6 hpf with continuous dTAG13 presence (Fig. 2a,
#1), which caused most embryos to arrest at the four-cell or eight-cell stage (Fig. 2b, #1, arrows), suggesting GABPA plays important roles before the four-cell stage. Since GABPA is highly
expressed at late two-cell stage (Fig. 1c,d), we asked whether GABPA regulates major ZGA. To this end, we treated the embryos with dTAG13 from 6 to 42 hpf and then washed out dTAG13 (Fig.
2a,b, #2). This treatment affected embryo development similarly to when dTAG13 is continuously present from 6 to 112 hpf. Moreover, GABPA degradation after major ZGA (42–112 hpf) resulted in
a much weaker phenotype (Fig. 2a,b, #3), suggesting that GABPA plays an important role in ZGA. To study GABPA’s role in regulating major ZGA, we treated the _Gabpa_dTAG/dTAG embryos with
dTAG13 from mid-zygote (6 hpf) to late two-cell stage (30 hpf) and collected embryos at 30 hpf for RNA-seq (Fig. 2c and Extended Data Fig. 2a), which revealed 589 downregulated and 78
upregulated genes (Fig. 2c). Gene Ontology (GO) analysis revealed that the downregulated genes are involved in processes such as ribosome biogenesis, ribosomal RNA processing and so on
(Extended Data Fig. 2b,c), explaining the embryo arrest phenotype. In contrast, the upregulated genes did not show GO term enrichment. Further analysis of the downregulated genes identified
104 major ZGA genes, indicating that GABPA has a role in activating these major ZGA genes (Fig. 2c and Supplementary Tables 1 and 2). To determine whether GABPA directly binds to and
regulates the downregulated genes, we performed low-input CUT&RUN on GABPA24,25. We first tested GABPA CUT&RUN in mouse ES cells and found the use of 500 ES cells generated similar
results as when using 20,000 cells (Extended Data Fig. 3a,b). We then performed GABPA CUT&RUN using late two-cell embryos (Extended Data Fig. 3b). Analysis of the CUT&RUN data
indicated that most GABPA binding peaks are located in the nucleosome-depleted regions of promoters (Fig. 2d and Extended Data Fig. 3c). Interestingly, the GABPA binding regions were
enriched for the GABPA motif but not the motifs of other murine ZGA regulators such as OBOX, DUX or NR5A226,27,28, suggesting direct GABPA binding at these regions (Extended Data Fig. 3c). A
clear GABPA binding signal around the transcription start sites (TSSs) of downregulated genes (449 out of 589), but not upregulated genes, was observed (Fig. 2e,f). Importantly, most (83
out of 104) downregulated major ZGA genes also showed direct GABPA promoter binding (Fig. 2f,g). The fact that GABPA degradation caused loss of promoter binding, resulting in the
downregulation of most GABPA direct targets (Extended Data Fig. 3d,e), including some major ZGA genes (Fig. 2c,g), supports that _Gabpa_ plays an important role in activating these major ZGA
genes by direct promoter binding. GABPA CONTROLS EPI SPECIFICATION BY ACTIVATING PLURIPOTENCY GENES Next, we asked whether GABPA has a role in the first cell lineage specification. To avoid
ZGA defect, we treated _Gabpa_dTAG/dTAG embryos with dTAG13 starting from the four-cell stage and collected early (84 hpf) and late (112 hpf) blastocysts for immunostaining (Fig. 3a).
Neither ICM nor TE cell numbers in early blastocysts were affected by the treatment (Fig. 3b,c), suggesting GABPA is not required for the first cell lineage specification. However, for late
blastocyst stage, the EPI, but not the PrE, cells were dramatically decreased by the treatment (Fig. 3d,e). RNA-seq analysis of morula embryos revealed a limited transcriptome change upon
the treatment (Extended Data Fig. 4a–c and Supplementary Table 3), consistent with minor role of GABPA from four-cell to morula embryos. To avoid a potential contribution from defects
between the four-cell stage and early blastocyst stage, we started the treatment in early blastocyst stage when the first cell lineage specification has already finished and then analysed
the effect on EPI and PrE at late blastocyst stage (Fig. 3f). A similar effect of this treatment to that of the treatment started at four-cell embryos was observed (Fig. 3g,h), indicating
that GABPA has a direct role in EPI specification. To understand how GABPA regulates EPI specification, we performed RNA-seq. To capture the earlier molecular changes that lead to EPI
defects, and to avoid potential confounding due to EPI and PrE cell ratio change, we collected ICM cells at the mid-blastocyst stage (100 hpf) when the EPI cell number had not yet shown a
difference between dimethyl sulfoxide (DMSO) and dTAG13 treatment (Extended Data Fig. 4d–f). Transcriptome analyses revealed 1,605 up- and 1,886 downregulated genes in response to GABPA
degradation (Fig. 3i, Extended Data Fig. 4g,h and Supplementary Table 4). Some pluripotency factors have already expressed at E3.5 ICM when GABPA degradation starts (Extended Data Fig. 4d);
although EPI could form at middle blastocyst, these EPI cells are defective in their transcriptome due to GABPA degradation (Fig. 3i). Importantly, 223 of the 1,886 (11.8%) downregulated
genes belong to the ICM genes, while 246 of the 1,605 (15.3%) upregulated genes belong to the TE genes (Fig. 3j). Further analysis revealed that 27% of the early ICM genes and 29.2% of the
late ICM genes were downregulated, while 21% of the early TE genes and 29.3% of the late TE genes were upregulated by GABPA degradation, indicating that GABPA plays an essential role in
pluripotency establishment (Fig. 3k and Extended Data Fig. 4i). We further found 46.8% of EPI genes were downregulated (Fig. 3l,m, Extended Data Fig. 4j and Supplementary Table 1). In
contrast, a much smaller percentage of PrE genes (1.6%) were downregulated, and importantly, most PrE marker genes, such as _Sox17_ and _Sox7_, did not show significant change (Fig. 3l,m and
Extended Data Fig. 4k), consistent with the observation that EPI, but not PrE, was affected upon GABPA degradation (Fig. 3d–h). Collectively, our data support that GABPA determines EPI but
not PrE specification by regulating EPI genes. GABPA REGULATES EPI GENE EXPRESSION BY PROMOTER AND DISTAL BINDING To understand how GABPA regulates the ICM genes in E4.5 embryos, we
performed GABPA CUT&RUN using E4.5 ICM cells (Extended Data Fig. 5a), which revealed that GABPA mainly occupies the promoter regions (Fig. 4a and Extended Data Fig. 5b). Motif analysis
revealed that these regions are enriched for the GABPA motif, but not motifs of other lineage regulators including OCT4, SOX2, NANOG and so on, indicating GABPA plays a direct role by
binding to these regions (Extended Data Fig. 5b). Integrative analyses of the GABPA CUT&RUN and RNA-seq data revealed that almost half of the downregulated genes (904 out of 1,886) were
bound by GABPA, which is consistent with its motif enrichment in these promoters (Fig. 4b). In contrast, much fewer upregulated genes are directly bound by GABPA (Fig. 4b). These results
indicate that GABPA directly binds to promoters to activate these genes in E4.5 ICM. By separating E4.5 ICM genes into early and late ICM genes, we found that GABPA mainly bound to the
promoters of early ICM genes (for example, _Tdgf1_, _Etv5_, _Dppa4_ and _Uhrf1_), but not late ICM genes (Fig. 4c,d). In addition to the promoter, GABPA also exhibited distal binding, which
were putative enhancer regions. We calculated the distance between the TSS of ICM/TE genes and their nearest distal GABPA peaks and found that the TSS of the ICM genes was closer to the
distal GABPA peaks than that of the TE genes (Fig. 4e), indicating a potential role of these distal GABPA bindings in regulating ICM genes. Indeed, we detected binding of GABPA to the known
super-enhancer of ICM gene _Sox2_29 (Extended Data Fig. 5c). To investigate whether GABPA would affect chromatin accessibility and/or promoter/enhancer activity, we performed ATAC–seq as
well as H3K27ac CUT&RUN in E4.5 ICM with or without GABPA degradation (Extended Data Fig. 5d). We found that GABPA degradation resulted in a widespread decrease of H3K27ac with little
effect on chromatin accessibility (Fig. 4f,g and Extended Data Fig. 5e). This result indicates that, while GABPA is not responsible for chromatin opening, it is important for the
transcriptional activity of the bound genes. Previous reports of the interaction between GABPA and p300 (refs. 30,31) in combination with the fact that GABPA degradation results in a
decrease in H3K27ac level suggest that GABPA may activate its targets by recruiting the acetyltransferase p300. GABPA REGULATES A COMMON SETS OF EPI GENES IN E4.5 ICM AND NAIVE ES CELLS
Since E4.5 ICM is composed of EPI and PrE cells, it is technically challenging to obtain pure EPI cells to evaluate the role of GABPA in regulating EPI gene expression. To overcome this
challenge, we used 2iES cells as they are believed to resemble the E4.5 EPI32. Since _Gabp_a KO affects ES cell survival33, we used the _Gabpa_dTAG/dTAG ES cells treated with dTAG13 to
evaluate the acute effect of GABPA degradation (Extended Data Fig. 6a). GABPA degradation was confirmed by western blot analysis (Fig. 1e) and immunostaining (Extended Data Fig. 6b). RNA-seq
revealed 2,265 downregulated genes and 1,563 upregulated genes in response to GABPA degradation for 24 h (Extended Data Fig. 6c,d and Supplementary Table 5). Importantly, GABPA CUT&RUN
analysis revealed that 1,238 (54.7%) of the downregulated genes have GABPA promoter binding (Fig. 5a and Extended Data Fig. 6e). Similar to that in E4.5 ICM, GABPA binding motif was enriched
in the GABPA peaks and its removal had little effect on ATAC–seq signals (Extended Data Fig. 6f,g). In contrast, the upregulated genes have much less GABPA direct promoter binding (Fig.
5a). Comparative analysis confirmed that GABPA binding in E4.5 ICM and ES cells was highly similar (Fig. 5b), suggesting that GABPA may regulate the same set of genes in ES cells and E4.5
ICM. Indeed, analysis of the differentially expressed genes (DEGs) in E4.5 ICM and ES cells revealed significant overlap with 933 commonly downregulated and 356 commonly upregulated genes in
response to GABPA degradation (Fig. 5c). These results indicate that GABPA regulates a similar set of genes in E4.5 ICM and 2iES cells. We further found that 26.3% EPI genes were
downregulated and 12.7% PrE genes were upregulated by GABPA degradation (Extended Data Fig. 6h), suggesting that GABPA plays an important role in pluripotency regulation in ES cells. Further
analysis of the downregulated ICM and EPI genes revealed that 80 ICM genes are in common (for example, _Spic_, _Utf1_, _Prdm14_ and _Utf1_) (Fig. 5d), including 60 early ICM genes and 20
late ICM genes (Extended Data Fig. 6i). The analysis also revealed 38 EPI genes in common (for example, _Nanog_, _Eras_ and _Pim2_) (Fig. 5e). Collectively, data from E4.5 ICM and 2iES cells
support the notion that GABPA plays a crucial role in regulating naive pluripotency both in vivo and in vitro. STEPWISE PLURIPOTENCY ESTABLISHMENT CONTROLLED BY TFAP2C/SOX2/GABPA The first
and second cell fate specification in mouse embryo occurs at E3.5 and E4.5 with pluripotency gene expression restricted within ICM and EPI, respectively34,35,36. However, the expression of
some ICM genes starts as early as the two-cell stage (Fig. 1b). A recent study revealed the role of the TFs NR5A2 and TFAP2C in activating ICM genes at the eight-cell stage10. However, the
expression of _Nr5a2_ and _Tfap2c_ is respectively silenced at E3.5 ICM and E4.5 EPI (Extended Data Fig. 7a), indicating that other TFs are responsible for the ICM gene activation at these
stages. Our data indicate that GABPA plays such a role for EPI gene activation at E4.5. To understand how GABPA participates in this process, we generated additional GABPA CUT&RUN
dataset in eight-cell and E3.5 ICM (Extended Data Fig. 7b,c). Comparative analysis of GABPA binding profiles at two-cell, eight-cell, E3.5 ICM and E4.5 ICM indicates that strong promoter
GABPA binding occurs at the two-cell stage and is maintained through E4.5 ICM (Fig. 6a and Extended Data Fig. 7d), which is consistent with the continuous expression of their target genes
(Extended Data Fig. 7e). Weak promoter binding at the two-cell stage became stronger at the E4.5 ICM stage (Fig. 6a). Increased GABPA binding to promoters of ICM genes at E4.5 ICM probably
contributes to GABPA’s stage-specific functions (Fig. 6a and Extended Data Fig. 7f). Next, we analysed the relationship between GABPA and NR5A2 by comparing their binding profiles at the
two-cell and eight-cell stages. Interestingly, GABPA tends to bind to promoters, while NR5A2 preferentially binds to putative enhancers (Fig. 6b), suggesting that they have distinct
mechanisms in gene regulation. We also compared the binding profiles of GABPA and TFAP2C at two-cell, eight-cell and E3.5 ICM and found that TFAP2C and GABPA co-occupy a portion of ICM genes
promoters at eight-cell and E3.5 ICM (Extended Data Fig. 7g), which may explain why GABPA degradation at these stages does not show phenotype. Given that TFAP2C is not expressed in E4.5 EPI
(Extended Data Fig. 7a)9, while GABPA binding is increased from E3.5 ICM to E4.5 ICM, GABPA may take over TFAP2C’s function on their commonly occupied ICM gene promoters (Fig. 6c,d). To
identify the commonly regulated genes taken over by GABPA, we compared the downregulated genes in GABPA-dTAG E4.5 ICM with the downregulated genes in _Tfap2c_ maternal-zygotic KO E3.5 ICM
and identified that 7 out of the 13 _Tfap2c_ KO downregulated ICM genes and all the 5 _Tfap2c_ KO downregulated EPI genes showed overlap (Extended Data Fig. 7h). Since _Tfap2c_
maternal-zygotic KO at the eight-cell stage showed more downregulated ICM and EPI genes than that in E3.5 ICM (Extended Data Fig. 7i), TFAP2C plays more important role in activating
pluripotency genes at the eight-cell than in E3.5 ICM, which is consistent with the observation that _Tfap2c_ expression is nearly undetectable at E3.5 ICM (Extended Data Fig. 7a)9. _Sox2_
starts to express at E3.5 ICM and plays an important role in regulating pluripotency (Extended Data Fig. 7a)8. We thus compared the binding profiles of GABPA and SOX2 in E3.5 ICM and E4.5
ICM. We found a global increase in GABPA binding concomitant with a global decrease in SOX2 binding, especially on promoters from E3.5 ICM to E4.5 ICM (Fig. 6e). Consistently, GABPA binding
on ICM gene promoters was enhanced, while SOX2 binding on ICM gene promoters was lost (Fig. 6f,g), indicating the regulation of ICM genes by GABPA was enhanced at E4.5 stage. To explore the
possibility that GABPA takes over the role of SOX2 in activating their commonly regulated ICM genes at E4.5, we compared the downregulated genes in GABPA-dTAG E4.5 ICM and _Sox2-_mzKO E3.5
ICM and found that 18 out of the 55 _Sox2-_KO downregulated ICM genes and 8 out of the 9 _Sox2-_KO downregulated EPI genes showed overlap (Extended Data Fig. 7j), supporting the notion that
GABPA takes over SOX2’s role on these ICM gene promoters in E4.5 ICM. To gain further support for this notion, we compared the downregulated genes of GABPA-dTAG E4.5 ICM with those of the
SOX2-dTAG 2iES cells. Although SOX2 degradation in 2iES cells downregulated only a small number of ICM (27) and EPI (5) genes, all the SOX2 downregulated EPI genes were also downregulated by
GABPA and more than half of the SOX2 downregulated ICM genes were also downregulated by GABPA in embryo (Fig. 6h). Three out of the 5 SOX2-dTAG downregulated EPI genes and 7 out of the 27
downregulated ICM genes were also downregulated by GABPA degradation in ES cells (Extended Data Fig. 7k). Collectively, these data support our notion that TFAP2C and SOX2 are responsible for
activating pluripotency genes at eight-cell and E3.5 ICM, respectively, while GABPA mainly activates pluripotency genes during E3.5 ICM to E4.5 EPI transition. GABPA takes over the role of
TFAP2C and SOX2 at the promoter of pluripotency genes in E4.5 EPI in addition to activating its unique targets. DISCUSSION ZGA and pluripotency acquisition are two of the most important
events during pre-implantation development. Understanding the molecular mechanisms underlying these events is not only important in the field of development but also critical for
regenerative medicine. Here, we identified and demonstrated that a minor ZGA factor GABPA not only regulates major ZGA but also plays a critical role in pluripotency establishment by
activating a large group of pluripotency genes in E4.5 EPI. Despite the observation that GABPA KO downregulates pluripotency factors _Nanog_ and _Oct4_ in ES cells33,37, its role as a key
pluripotency regulator was not recognized due to the lack of direct genome binding data. Additionally, its KO lethality phenotype before blastocyst formation21 prevented its role in
pluripotency in vivo from being addressed using the conventional KO mouse model. In this study, using the dTAG system, we reveal the developmental stage-specific function of GABPA during
mouse pre-implantation development. Our data support that GABPA plays a non-dispensable role in ZGA and serves as a master regulator of pluripotency establishment at E4.5 EPI. The
identification of TFs important for mammalian ZGA has been a hot topic in the past several years. Previous studies have been mainly focused on maternal factors that regulate mouse ZGA such
as OBOX26, NR5A228, DUX27, NFYA38 and KLF1739, while the contributions of zygotic early transcribed genes are neglected, although published work has shown that minor ZGA is required for
major ZGA in mice40. Here, we provide evidence demonstrating that the minor ZGA factor GABPA regulates a subset of major ZGA genes and pre-implantation development by directly binding to the
promoter of 83 major ZGA genes to activate their transcription (Fig. 2g). Our study indicates that minor ZGA gene product can regulate major ZGA by directly binding to and activating major
ZGA. Further studies of the other minor ZGA genes are warranted to fully understand the role of minor ZGA genes in regulating major ZGA and pre-implantation development. Another important
finding of this study is that we identify GABPA as a key TF driving the E4.5 EPI specification. We showed that GABPA degradation affects EPI, but not PrE, formation (Fig. 3d–h), which could
be explained by GABPA’s role in activating pluripotency genes including _Nanog_ and _Sox2_ (Fig. 3m). Different from _Nanog_ and _Sox2_, which are exclusively expressed in EPI, _Gabpa_ is
expressed in both EPI and PrE. This expression pattern raises an intriguing question about why GABPA selectively activates EPI genes, but not PrE genes (Fig. 3l,m). One possibility is that
binding of GABPA to the pluripotency gene promoters requires another factor that is expressed only in EPI. Alternatively, PrE may express a protein that can mask GABPA binding to promoters.
Future studies should confirm or refute these possibilities. Establishment of pluripotency is a continuous and complex process. Although the expression of pluripotency genes such as _Nanog_,
_Sox2_ and _Oct4_ are critical for maintaining pluripotency41,42,43,44, their role in pluripotency establishment has not been shown. At eight-cell and E3.5 ICM, TFs such as NR5A2, TFAP2C
and SOX2 are believed to modulate the activity of these pluripotency genes8,9. They are unlikely to have a major role in EPI specification as they either are not expressed in EPI or they
only occupy the promoters of very few pluripotency genes in EPI. Our data indicate that GABPA can fulfil such a role as it regulates 46.8% and 26.3% of EPI genes in E4.5 and 2iES cells,
respectively (Fig. 6i). Although the lack of a dTAG mouse model for SOX2 prevented a direct comparison of the role of GABPA and SOX2 in EPI formation, a comparison of dTAG13-mediated
degradation of GABPA or SOX2 in 2iES cells showed a clear difference as GABPA degradation downregulated many more ICM genes and EPI genes than did SOX2 degradation (Fig. 6i). Our study,
together with previous studies, supports a stepwise model for naive pluripotency establishment (Fig. 6j). At the eight-cell stage, TFAP2C binds to gene promoters and enhancers to active
pluripotency genes. At E3.5, with the decrease of TFAP2C expression in ICM, its role in pluripotency gene regulation decreases, while SOX2 starts to function. By E4.5, TFAP2C is no longer
expressed in ICM (Extended Data Fig. 7a)9, and the binding of SOX2 to the promoters of ICM genes also disappeared, while GABPA now occupies and activates almost half of the pluripotency
genes in EPI. Our study thus not only identifies GABPA as an important TF for ZGA but also demonstrates its crucial role as a master regulator of naive pluripotency in E4.5 embryos. METHODS
ANIMALS All experiments were conducted in accordance with the National Institute of Health Guide for Care and Use of Laboratory Animals and approved by the Institutional Animal Care and Use
Committee of Boston Children’s Hospital and Harvard Medical School (protocol number IS00000270-6). All mice were kept under specific pathogen-free conditions within an environment controlled
for temperature (20–22 °C) and humidity (40–70%), and were subjected to a 12 h light/dark cycle. The generation of _Gabpa_-dTAG knock-in mice was as described previously with some
modifications23. Briefly, two-cell embryos (20 hpf) were injected with _Gabpa_ donor DNA (30 ng μl−1), Cas9 mRNA (100 ng μl−1) and sgRNA (single guide RNA) (50 ng μl−1) using a Piezo
impact-driven micromanipulator (Primer Tech). Then, two-cell embryos were incubated in KSOM (potassium simplex optimized medium) for 2 h before transfer into oviducts of pseudo-pregnant
Institute of Cancer Research (ICR) strain mothers (Charles River). F0 chimera mice were backcrossed with wild-type C57BL/6J mice for at least two generations. Genotyping was performed using
mouse tail lysed in lysis buffer (50 mM Tris–HCl, 0.5% Triton and 400 μg ml−1 Proteinase K) at 55 °C overnight. For F0 and F1 mice genotyping, the primers outside the homology arm are used.
For genotyping of F2 and beyond, the inner primers are used. The primers are listed in Supplementary Table 6. Droplet digital PCR (ddPCR) was used for detecting the copy number of
_Gabpa_-dTAG knock-in alleles in F1 mice. Briefly, 250 ng purified DNA templates were digested by incubation with Haelll Enzyme (NEB) at 37 °C for 1 h and then inactivated at 80 °C for 5
min. A final 30 ng of DNA was used as the templates for PCR. _Fkbp_ was used for knock-in detecting, and _mRPP30_ was used as a control. The primers are included in Supplementary Table 6.
Only the mice with a single _Fkbp_ copy (Supplementary Table 7) were used for further mating. MES CELL CULTURE AND ESTABLISHMENT OF _GABPA_ DTAG/DTAG CELL LINE The laboratory-maintained
ES-E14 cells45 were cultured on 0.1% gelatin-coated plates with 2i/LIF (MEKi/GSK3i/leukemia inhibitory factor) condition. Cells were grown in Dulbecco’s modified Eagle medium (Gibco,
11960069), supplemented with 15% foetal bovine serum (Sigma-Aldrich, F6178), 2 mM GlutaMAX (Gibco, 35050061), 1 mM sodium pyruvate (Gibco, 11360), 1× MEM NEAA (minimum essential medium
nonessential amino acids) (Gibco, 11140050), 0.084 mM 2-mercaptoethanol (Gibco, 21985023), 1 mM sodium pyruvate (Gibco, 11360070), 100 U ml−1 penicillin–streptomycin (Gibco, 15140122), 1,000
IU ml−1 LIF (Millipore, ESG1107), 0.5 μM PD0325901 (Tocris, 4192) and 3 μM CHIR99021 (Tocris, 4423). To establish _Gabpa_dTAG/dTAG cell line, _Gabpa_-HAL-FKBPF36V-2xHA-HAR and px330 were
transfected into mouse (m)ES cells with Lipofectamine 2000 (Thermo, 11668030). Twenty-four hours later, cells were selected with puromycin (Gibco, A1113803) for another 48 h. Then, cells
were cultured in puromycin-free medium for 1 week. Single clones were picked for genotyping and further analysis. IN VITRO FERTILIZATION AND EMBRYO CULTURE Female mice (7–8 weeks) were
superovulated through an initial injection of 7.5 IU pregnant mare serum gonadotropin (BioVendor, RP1782725000), followed 48 h later with a 7.5 IU injection of human chorionic gonadotropin
(Sigma, C1063). Oocyte–cumulus complexes (OCCs) were collected 14 h post human chorionic gonadotropin injection. Sperm was collected from the cauda epididymis of adult male mice (8–12 weeks)
1 h before OCC collection. The sperm suspension was capacitated for 1 h in 200 μl human tubal fluid (HTF) medium (Millipore, MR-070-D). Subsequently, OCCs were exposed to spermatozoa for a
6 h incubation. The time when sperm was added to OCCs was considered as 0 hpf. Two-nuclear zygotes were cultured in the KSOM medium (Millipore, MR-106-D) under a humidified atmosphere of 5%
CO2 at 37 °C for further development. WESTERN BLOT A total of 1.5 × 106 cells were lysed in 100 µl RIPA lysis buffer and incubated on ice for 30 min. Ninety microlitres of supernatant was
mixed with 12.5 µl 5× loading buffer, and heated at 98 °C for 15 min. Samples were run on NuPAGE 4–12% gel (Invitrogen, NP0322BOX) and transferred onto polyvinylidene fluoride transfer
membrane. Primary antibodies used included anti-GABPA (1:4,000, Proteintech), anti-HA (1:1,000, CST) and anti-β-actin (1:5,000, CST, #4967). Secondary antibodies used included goat
anti-Rabbit IgG (H + L) superclonal secondary antibody-HRP (Thermo Scientific, A27036, 1:2,000) and goat anti-mouse IgG (H + L) secondary antibody-HRP (Thermo Fisher Scientific, 31430,
1:2,000). Protein bands were detected with an enhanced chemiluminescence (ECL) kit (Thermo Fisher Scientific, 32209) and imaged by Tanon 4600SF Imaging System (Tanon). IMMUNOSTAINING AND
CONFOCAL MICROSCOPE Embryos were fixed with 4% paraformaldehyde/0.5% Triton for 20 min, followed by three times of washing with phosphate-buffered saline/0.1% Triton, then blocked in
phosphate-buffered saline/1% bovine serum albumin/0.01% Triton for 1 h. Embryos were incubated overnight at 4 °C with primary antibodies: anti-GABPA (1:200, Proteintech, 21542-1-AP, lot no.
00018047), anti-HA (1:200, CST, 2367S), anti-GATA4 (1:200, R&D Systems, MAB2606-SP), anti-NANOG (1:200, Abcam, ab80892) and anti-CDX2 (1:500, R&D Systems, AF3665-SP). Secondary
antibodies used included donkey anti-goat IgG (H + L) secondary antibody, Alexa Fluor 647 (Thermo Scientific, A-21447, 1:500), donkey anti-rabbit IgG (H + L) secondary antibody, Alexa Fluor
488 (Thermo Scientific, A-21206, 1:500) and Donkey anti-mouse IgG Secondary Antibody, Alexa Fluor 568 (Fisher Scientific, A10037, 1:500). After three times of washing, the embryos were
incubated with secondary antibodies at room temperature (RT). DNA was stained with 10 μg ml−1 Hoechst 33342 (Sigma). The confocal microscope (Zeiss, LSM800) was used for fluorescence
detection. DTAG13 TREATMENT dTAG13 (Tocris, 6605) was reconstituted in DMSO to a 5 mM stock. For _Gabpa_dTAG/dTAG mES cell treatment, dTAG13 was diluted in mES cell culture medium to 0.5 μM.
For _Gabpa_dTAG/dTAG embryo treatment, dTAG13 was diluted in KSOM to 1 μM. Embryos were washed with KSOM with dTAG13 at least three times, then cultured in KSOM with dTAG13 for further
development. CUT&RUN, ATAC AND RNA-SEQ LIBRARY PREPARATION AND SEQUENCING For mES cell CUT&RUN with more than 10,000 cells were resuspended in 50 μl washing buffer (20 mM HEPES pH
7.5, 150 mM NaCl, 0.5 mM spermidine and 1× protease inhibitor) with activated Concanavalin A magnetic beads (Polysciences, 86057-3) for 10 min at RT, then samples were incubated with
anti-GABPA (1:40, Proteintech, 21542-1-AP, lot#00018047; note that this is the only lot that worked in CUT&RUN in our hands) overnight at 4 °C. For low-input mES cells and embryo
CUT&RUN, some modifications were made. Briefly, mES cells, zona-free embryos or isolated ICM were resuspended in 50 μl washing buffer with activated Concanavalin A magnetic beads for 10
min at RT, then samples were fixed with 1% formaldehyde for 1 min. After three washes with washing buffer, samples were incubated with anti-GABPA overnight at 4 °C. Samples were incubated
with 2.8 ng μl−1 pA-MNase (home-made) for 2 h at 4 °C. Subsequently, samples were incubated with 200 μl pre-cooled 0.5 μM CaCl2 for 20 min at 4 °C and quenched by adding 23 μl 10× stop
buffer (1,700 mM NaCl, 20 mM EGTA, 100 mM EDTA, 0.02% digitonin, 250 µg ml−1 glycogen and 250 µg ml−1 RNase A). DNA fragments were released by incubation at 37 °C for 15 min. For both fixed
and unfixed cells, 2.5 μl 10% SDS and 2.5 μl 20 mg ml−1 Proteinase K (Thermo Fisher) was added and incubated at 55 °C for at least 1 h for reverse crosslinking. DNA was extracted by
phenol–chloroform followed by ethanol precipitation. The subsequent procedure was the same as described above. Sequencing libraries were prepared with NEBNext Ultra II DNA library
preparation kit for Illumina (New England Biolabs, E7645S). ATAC–seq was performed as previously described with some modifications46. Briefly, ES cells and isolated ICM were digested with
adapter-loaded Tn5 for 15 min at 37 °C, and stopped by stop buffer (100 mM Tris pH 8.0, 100 mM NaCl, 40 µg ml−1 Proteinase K and 0.4% SDS) and incubated overnight at 55 °C. Five microlitres
of 25% Tween-20 was added to quench SDS. Sequencing libraries were prepared with NEBNext High-Fidelity 2× PCR Master Mix (NEB, M0541S). For RNA-seq, fresh ES cells or embryos were collected.
Reverse transcription and complementary DNA amplification were performed with SMART-Seq v4 Ultra Low Input RNA Kit (Clontech, 634890), followed by cDNA fragmentation, adaptor ligation and
amplification using Nextera XT DNA Sample Preparation Kit (Illumina, FC-131-1024). All libraries were sequenced by NextSeq 550 system (Illumina) with paired-ended 75 bp reads (Supplementary
Table 8). IMMUNOSURGERY ICMs were isolated as previously described47. Briefly, blastocysts at E3.5 or E4.5 stages were collected by removing the zona pellucida with Acidic Tyrode’s solution
(Millipore). Embryos were then treated with anti-mouse serum antibody (Sigma-Aldrich, M5774-2ML, 1:5 dilution in KSOM) for 30 min at 37 °C. After washing three times with KSOM, embryos were
treated with guinea pig complement (Millipore, 1:5 dilution in KSOM) for another 20 min at 37 °C. Then, the TE cells were removed by a glass pipette (the inner diameter is around 40–50 μm).
E4.5 ICM refers to the mixture of EPI and PrE cells after removing TE cells with immunosurgery. RNA-SEQ DATA ANALYSIS The raw sequencing reads were trimmed with Trimmomatic48 (v0.39) to
remove sequencing adaptors. Then, the reads were mapped to GRCm38 genome using STAR49 (v2.7.8a). Gene expression levels were quantified with RSEM50 (v1.3.1). To identify differentially
expression genes, the DESeq251 (v1.32.0) package in R was used. The significantly differentially expressed genes were called with an adjusted _P_-value cut-off of 0.05, fold change cut-off
of 2 and mean FPKM (fragments per kilobase of transcript per million mapped reads) cut-off of 1. GO enrichment was performed using R package clusterProfiler52. Gene Set Enrichment Analysis
(GSEA) was performed using R clusterProfiler52 and enrichplot. CUT&RUN DATA ANALYSIS The raw reads were trimmed with Trimmomatic48 (v0.39) to remove sequencing adaptors, then mapped to
GRCm38 reference genome using bowtie253 (v2.4.2). PCR duplicates were removed with Picard MarkDuplicates (v2.23.4). Reads with mapping quality less than 30 were removed. The mapped reads
were further filtered to retain only proper paired reads with fragment length between 10 and 120. Peaks were called with MACS254 (v2.2.7.1). Reproducible peaks were generated with the
irreproducible discovery rate (IDR) framework55 using two replicates, with IDR threshold of 0.05. For E4.5 ICM and ES cells, we further filtered the peaks to keep the ones with _q_ value
≤10−30 to remove weak peaks. The signal tracks were generated with deeptools56 bamCoverage (v3.5.1) with bin size of 1 and normalized by CPM (counts per million). For _z_-score-normalized
signal tracks, we first used bamCoverage with bin size of 100 to generate FPKM signals, then used a customized script to calculate the _z_ score of each bin. For late two-cell and eight-cell
GABPA ultralow-input CUT&RUN data, we noticed low mapping rates of the raw data. Further examination of the unmapped reads suggested they were environmental DNA from human, bacteria,
vectors and so on, and due to the ultralow-input cells and small number of GABPA binding regions, the ratio of mapped reads from GABPA-bound DNA versus unmapped reads arising from
environmental DNA was low. However, this would not affect the identification of GABPA peaks, since (1) the discarded reads were unmappable to mouse reference genome and (2) these peaks
disappeared upon GABPA degradation. The peaks were annotated with R package ChIPseeker57. Peaks within −1,000 to +500 around the TSS were considered as promoter peaks. The heatmaps of
binding profiles were calculated with deeptools56 computeMatrix (v3.5.1) using bigwig signal tracks as input and bin size of 10, and visualized in R with packages profileplyr and
EnrichedHeatmap58. ATAC–SEQ DATA ANALYSIS ATAC–seq data were analysed with the ENCODE59 ATAC–seq pipeline with default parameters (v2.1.2, https://github.com/ENCODE-DCC/atac-seq-pipeline).
MOTIF ENRICHMENT ANALYSIS Motif enrichment analysis was performed with HOMER60 (v4.11) findMotifsGenome.pl with mm10 reference and parameter size 200, using peaks file as input. MOTIF
OCCURRENCE ANALYSIS Motif occurrence analysis was performed with HOMER60 (v4.11) annotatePeaks.pl with parameters ‘mm10 -size -2000,2000 -hist 20 -ghist’ for peak regions and parameters
‘mm10 -size -500,500 -hist 20 -ghist’ for regions around gene TSSs. The motif files were download from JASPAR database61 and manually converted to HOMER motif format. We used the log odds
detection threshold of 6.0 for all TFs we analysed. The motif occurrence matrix was visualized in R with package EnrichedHeatmap58. The JASPAR motif IDs for the TFs we analysed were as
follows: GABPA, MA0062.2; OBOX, PH0121.1; DUX, MA0611.1; NFYA, MA0060.1; NR5A2, MA0505.1; OCT4, MA1115.1; SOX2, MA0143.1; NANOG, MA2339.1; TFAP2C, MA0524.2; GATA3, MA0037.4. IDENTIFICATION
OF ICM/TE GENES ICM/TE genes were identified using bulk RNA-seq data of E3.5 ICM and E3.5 TE from Zhang et al.18. The DESeq251 (v1.32.0) package in R was used to find the DEGs between ICM
and TE, with an adjusted _P_-value cut-off of 0.05 and fold change cut-off of 2. Genes with eight-cell FPKM ≥1 were defined as early ICM/TE genes, while genes with eight-cell FPKM <1 were
defined as late ICM/TE genes. IDENTIFICATION OF EPI/PRE GENES EPI/PrE genes were identified using single-cell RNA-seq data of E4.5 embryos from Mohammed et al.62. The R package Seurat63
(v5.0.1) function FindMarkers was used to identify the DEGs between E4.5 EPI and E4.5 PrE, with an adjusted _P_-value cut-off of 0.05, fold change cut-off of 4 and cell expression percentage
cut-off of 0.8. Genes with expression in at least 50% E3.5 single cells were considered as early EPI/PrE genes, while the others were considered as late EPI/PrE genes. STATISTICS AND
REPRODUCIBILITY Student’s _t_-tests for graph analysis were performed with Microsoft Excel (2016). Individual data points are shown as dots in the figure panels involving the Student’s
_t_-test. Data distribution was assumed to be normal, but this was not formally tested. For the immunofluorescence and western blot experiments, at least three independent repetitions were
performed with consistent results, and representative data were presented. _P_ values < 0.05 were considered statistically significant. No statistical methods were used to pre-determine
sample sizes, but our sample sizes are similar to or greater than those reported in previous publications8. No data were excluded from the analyses. The experiments were not randomized. Data
collection and analysis were not performed blind to the conditions of the experiments. REPORTING SUMMARY Further information on research design is available in the Nature Portfolio
Reporting Summary linked to this article. DATA AVAILABILITY Sequencing data that support the findings of this study have been deposited in the Gene Expression Omnibus (GEO) under accession
code GSE263171. Public data used in this study: RNA-seq of mouse MII oocyte to eight-cell17: GSE71434. RNA-seq of mouse E3.5 ICM and TE18: GSE76505. RNA-seq of mouse E4.5 TE9: GSE216256.
Single-cell RNA-seq of mouse E4.5 EPI and PrE64: GSE159030. Single-cell RNA-seq of mouse early embryos19: GSE45719. Single-cell RNA-seq of mouse E3.5 and E4.5 embryos62: GSE100597. ATAC–seq
of mouse early embryos16: GSE66390. NR5A2 binding in mouse embryos10: GSE229740. TFAP2C binding in mouse embryos9: GSE216256. SOX2 binding in mouse embryos8: GSE203194. Source data are
provided with this paper. All other data supporting the findings of this study are available from the corresponding author on reasonable request. REFERENCES * Schulz, K. N. & Harrison,
M. M. Mechanisms regulating zygotic genome activation. _Nat. Rev. Genet._ 20, 221–234 (2019). CAS PubMed PubMed Central Google Scholar * Fu, X., Zhang, C. & Zhang, Y. Epigenetic
regulation of mouse preimplantation embryo development. _Curr. Opin. Genet. Dev._ 64, 13–20 (2020). CAS PubMed PubMed Central Google Scholar * Zernicka-Goetz, M., Morris, S. A. &
Bruce, A. W. Making a firm decision: multifaceted regulation of cell fate in the early mouse embryo. _Nat. Rev. Genet._ 10, 467–477 (2009). CAS PubMed Google Scholar * Chen, Q., Shi, J.,
Tao, Y. & Zernicka-Goetz, M. Tracing the origin of heterogeneity and symmetry breaking in the early mammalian embryo. _Nat. Commun._ 9, 1819 (2018). PubMed PubMed Central Google
Scholar * Rossant, J. Genetic control of early cell lineages in the mammalian embryo. _Annu. Rev. Genet._ 52, 185–201 (2018). CAS PubMed Google Scholar * Pera, M. F. & Rossant, J.
The exploration of pluripotency space: charting cell state transitions in peri-implantation development. _Cell Stem Cell_ 28, 1896–1906 (2021). CAS PubMed Google Scholar * Boroviak, T.,
Loos, R., Bertone, P., Smith, A. & Nichols, J. The ability of inner-cell-mass cells to self-renew as embryonic stem cells is acquired following epiblast specification. _Nat. Cell Biol._
16, 516–528 (2014). CAS PubMed PubMed Central Google Scholar * Li, L. et al. Multifaceted SOX2–chromatin interaction underpins pluripotency progression in early embryos. _Science_ 382,
eadi5516 (2023). CAS PubMed Google Scholar * Li, L. et al. Lineage regulators TFAP2C and NR5A2 function as bipotency activators in totipotent embryos. _Nat. Struct. Mol. Biol._ 31,
950–963 (2024). CAS PubMed Google Scholar * Lai, F. et al. NR5A2 connects zygotic genome activation to the first lineage segregation in totipotent embryos. _Cell Res._ 33, 952–966 (2023).
CAS PubMed PubMed Central Google Scholar * Wicklow, E. et al. HIPPO pathway members restrict SOX2 to the inner cell mass where it promotes ICM fates in the mouse blastocyst. _PLoS
Genet._ 10, e1004618 (2014). PubMed PubMed Central Google Scholar * Silva, J. et al. Nanog is the gateway to the pluripotent ground state. _Cell_ 138, 722–737 (2009). CAS PubMed PubMed
Central Google Scholar * Nishimura, K., Fukagawa, T., Takisawa, H., Kakimoto, T. & Kanemaki, M. An auxin-based degron system for the rapid depletion of proteins in nonplant cells.
_Nat. Methods_ 6, 917–922 (2009). CAS PubMed Google Scholar * Nabet, B. et al. The dTAG system for immediate and target-specific protein degradation. _Nat. Chem. Biol._ 14, 431–441
(2018). CAS PubMed PubMed Central Google Scholar * Abuhashem, A., Lee, A. S., Joyner, A. L. & Hadjantonakis, A. K. Rapid and efficient degradation of endogenous proteins in vivo
identifies stage-specific roles of RNA Pol II pausing in mammalian development. _Dev. Cell_ 57, 1068–1080 e1066 (2022). CAS PubMed PubMed Central Google Scholar * Wu, J. et al. The
landscape of accessible chromatin in mammalian preimplantation embryos. _Nature_ 534, 652–657 (2016). CAS PubMed Google Scholar * Zhang, B. et al. Allelic reprogramming of the histone
modification H3K4me3 in early mammalian development. _Nature_ 537, 553–557 (2016). CAS PubMed Google Scholar * Zhang, Y. et al. Dynamic epigenomic landscapes during early lineage
specification in mouse embryos. _Nat. Genet._ 50, 96–105 (2018). CAS PubMed Google Scholar * Deng, Q., Ramskold, D., Reinius, B. & Sandberg, R. Single-cell RNA-seq reveals dynamic,
random monoallelic gene expression in mammalian cells. _Science_ 343, 193–196 (2014). CAS PubMed Google Scholar * Rosmarin, A. G., Resendes, K. K., Yang, Z., McMillan, J. N. &
Fleming, S. L. GA-binding protein transcription factor: a review of GABP as an integrator of intracellular signaling and protein-protein interactions. _Blood Cells Mol. Dis._ 32, 143–154
(2004). CAS PubMed Google Scholar * Ristevski, S. et al. The ETS transcription factor GABPα is essential for early embryogenesis. _Mol. Cell. Biol._ 24, 5844–5849 (2004). CAS PubMed
PubMed Central Google Scholar * Liu, N. Q. et al. WAPL maintains a cohesin loading cycle to preserve cell-type-specific distal gene regulation. _Nat. Genet._ 53, 100–109 (2021). CAS
PubMed Google Scholar * Gu, B., Posfai, E. & Rossant, J. Efficient generation of targeted large insertions by microinjection into two-cell-stage mouse embryos. _Nat. Biotechnol._ 36,
632–637 (2018). CAS PubMed Google Scholar * Skene, P. J. & Henikoff, S. An efficient targeted nuclease strategy for high-resolution mapping of DNA binding sites. _eLife_ 6, e21856
(2017). PubMed PubMed Central Google Scholar * Prakash, S. A. & Barau, J. Chromatin profiling in mouse embryonic germ cells by CUT&RUN. _Methods Mol. Biol._ 2214, 253–264 (2021).
CAS PubMed Google Scholar * Ji, S. et al. OBOX regulates mouse zygotic genome activation and early development. _Nature_ 620, 1047–1053 (2023). CAS PubMed PubMed Central Google Scholar
* Chen, Z. & Zhang, Y. Loss of DUX causes minor defects in zygotic genome activation and is compatible with mouse development. _Nat. Genet._ 51, 947–951 (2019). CAS PubMed PubMed
Central Google Scholar * Gassler, J. et al. Zygotic genome activation by the totipotency pioneer factor Nr5a2. _Science_ 378, 1305–1315 (2022). CAS PubMed Google Scholar * Zhou, H. Y.
et al. A Sox2 distal enhancer cluster regulates embryonic stem cell differentiation potential. _Genes Dev_ 28, 2699–2711 (2014). PubMed PubMed Central Google Scholar * Bannert, N., Avots,
A., Baier, M., Serfling, E. & Kurth, R. GA-binding protein factors, in concert with the coactivator CREB binding protein/p300, control the induction of the interleukin 16 promoter in T
lymphocytes. _Proc. Natl Acad. Sci. USA_ 96, 1541–1546 (1999). CAS PubMed PubMed Central Google Scholar * Bush, T. S., St Coeur, M., Resendes, K. K. & Rosmarin, A. G. GA-binding
protein (GABP) and Sp1 are required, along with retinoid receptors, to mediate retinoic acid responsiveness of CD18 (beta 2 leukocyte integrin): a novel mechanism of transcriptional
regulation in myeloid cells. _Blood_ 101, 311–317 (2003). CAS PubMed Google Scholar * Boroviak, T. et al. Lineage-specific profiling delineates the emergence and progression of naive
pluripotency in mammalian embryogenesis. _Dev. Cell_ 35, 366–382 (2015). CAS PubMed PubMed Central Google Scholar * Ueda, A., Akagi, T. & Yokota, T. GA-binding protein alpha is
involved in the survival of mouse embryonic stem cells. _Stem Cells_ 35, 2229–2238 (2017). CAS PubMed Google Scholar * Lim, H. Y. G. & Plachta, N. Cytoskeletal control of early
mammalian development. _Nat. Rev. Mol. Cell Biol._ 22, 548–562 (2021). CAS PubMed Google Scholar * Zhu, M. & Zernicka-Goetz, M. Principles of self-organization of the mammalian
embryo. _Cell_ 183, 1467–1478 (2020). CAS PubMed PubMed Central Google Scholar * Zhang, H. T. & Hiiragi, T. Symmetry breaking in the mammalian embryo. _Annu. Rev. Cell Dev. Biol._
34, 405–426 (2018). CAS PubMed Google Scholar * Kinoshita, K. et al. GABPα regulates Oct-3/4 expression in mouse embryonic stem cells. _Biochem. Biophys. Res. Commun._ 353, 686–691
(2007). CAS PubMed Google Scholar * Lu, F. et al. Establishing chromatin regulatory landscape during mouse preimplantation development. _Cell_ 165, 1375–1388 (2016). CAS PubMed PubMed
Central Google Scholar * Hu, Y. et al. Maternal KLF17 controls zygotic genome activation by acting as a messenger for RNA Pol II recruitment in mouse embryos. _Dev. Cell_ 59, 613–626 e616
(2024). CAS PubMed Google Scholar * Abe, K. I. et al. Minor zygotic gene activation is essential for mouse preimplantation development. _Proc. Natl Acad. Sci. USA_ 115, E6780–E6788
(2018). CAS PubMed PubMed Central Google Scholar * Ding, J., Xu, H., Faiola, F., Ma’ayan, A. & Wang, J. Oct4 links multiple epigenetic pathways to the pluripotency network. _Cell
Res_ 22, 155–167 (2012). CAS PubMed Google Scholar * Murakami, K. et al. NANOG alone induces germ cells in primed epiblast in vitro by activation of enhancers. _Nature_ 529, 403–407
(2016). CAS PubMed PubMed Central Google Scholar * Surani, M. A., Hayashi, K. & Hajkova, P. Genetic and epigenetic regulators of pluripotency. _Cell_ 128, 747–762 (2007). CAS PubMed
Google Scholar * Masui, S. et al. Pluripotency governed by Sox2 via regulation of Oct3/4 expression in mouse embryonic stem cells. _Nat. Cell Biol._ 9, 625–635 (2007). CAS PubMed Google
Scholar * Fu, X., Djekidel, M. N. & Zhang, Y. A transcriptional roadmap for 2C-like-to-pluripotent state transition. _Sci. Adv._ 6, eaay5181 (2020). CAS PubMed PubMed Central Google
Scholar * Zhang, C. et al. The chromatin remodeler Snf2h is essential for oocyte meiotic cell cycle progression. _Genes Dev._ 34, 166–178 (2020). CAS PubMed PubMed Central Google
Scholar * Chen, Z., Djekidel, M. N. & Zhang, Y. Distinct dynamics and functions of H2AK119ub1 and H3K27me3 in mouse preimplantation embryos. _Nat. Genet._ 53, 551–563 (2021). CAS
PubMed PubMed Central Google Scholar * Bolger, A. M., Lohse, M. & Usadel, B. Trimmomatic: a flexible trimmer for Illumina sequence data. _Bioinformatics_ 30, 2114–2120 (2014). CAS
PubMed PubMed Central Google Scholar * Dobin, A. et al. STAR: ultrafast universal RNA-seq aligner. _Bioinformatics_ 29, 15–21 (2013). CAS PubMed Google Scholar * Li, B. & Dewey, C.
N. RSEM: accurate transcript quantification from RNA-seq data with or without a reference genome. _BMC Bioinf._ 12, 323 (2011). CAS Google Scholar * Love, M. I., Huber, W. & Anders,
S. Moderated estimation of fold change and dispersion for RNA-seq data with DESeq2. _Genome biology_ 15, 550 (2014). PubMed PubMed Central Google Scholar * Yu, G., Wang, L. G., Han, Y.
& He, Q. Y. clusterProfiler: an R package for comparing biological themes among gene clusters. _OMICS_ 16, 284–287 (2012). CAS PubMed PubMed Central Google Scholar * Langmead, B.
& Salzberg, S. L. Fast gapped-read alignment with Bowtie 2. _Nat. Methods_ 9, 357–359 (2012). CAS PubMed PubMed Central Google Scholar * Zhang, Y. et al. Model-based analysis of
ChIP-seq (MACS). _Genome Biol._ 9, R137 (2008). PubMed PubMed Central Google Scholar * Landt, S. G. et al. ChIP-seq guidelines and practices of the ENCODE and modENCODE consortia. _Genome
Res._ 22, 1813–1831 (2012). CAS PubMed PubMed Central Google Scholar * Ramirez, F. et al. deepTools2: a next generation web server for deep-sequencing data analysis. _Nucleic Acids
Res._ 44, W160–W165 (2016). CAS PubMed PubMed Central Google Scholar * Yu, G., Wang, L. G. & He, Q. Y. ChIPseeker: an R/Bioconductor package for ChIP peak annotation, comparison and
visualization. _Bioinformatics_ 31, 2382–2383 (2015). CAS PubMed Google Scholar * Gu, Z., Eils, R., Schlesner, M. & Ishaque, N. EnrichedHeatmap: an R/Bioconductor package for
comprehensive visualization of genomic signal associations. _BMC Genomics_ 19, 234 (2018). PubMed PubMed Central Google Scholar * Encode Project Consortiumet al. Expanded encyclopaedias
of DNA elements in the human and mouse genomes. _Nature_ 583, 699–710 (2020). Google Scholar * Heinz, S. et al. Simple combinations of lineage-determining transcription factors prime
cis-regulatory elements required for macrophage and B cell identities. _Mol. Cell_ 38, 576–589 (2010). CAS PubMed PubMed Central Google Scholar * Castro-Mondragon, J. A. et al. JASPAR
2022: the 9th release of the open-access database of transcription factor binding profiles. _Nucleic Acids Res._ 50, D165–D173 (2022). CAS PubMed Google Scholar * Mohammed, H. et al.
Single-cell landscape of transcriptional heterogeneity and cell fate decisions during mouse early gastrulation. _Cell Rep._ 20, 1215–1228 (2017). CAS PubMed PubMed Central Google Scholar
* Hao, Y. et al. Dictionary learning for integrative, multimodal and scalable single-cell analysis. _Nat. Biotechnol._ 42, 293–304 (2024). CAS PubMed Google Scholar * Stirparo, G. G. et
al. OCT4 induces embryonic pluripotency via STAT3 signaling and metabolic mechanisms. _Proc. Natl Acad. Sci. USA_ 118, e2008890118 (2021). CAS PubMed PubMed Central Google Scholar
Download references ACKNOWLEDGEMENTS We thank Y. Hagihara and Q. Yang for their valuable discussions on the project and comments on the manuscript. This project was supported by the National
Institutes of Health (NIH; R01HD092465) and the Howard Hughes Medical Institute (HHMI). Y.Z. is an investigator of the Howard Hughes Medical Institute. This Article is subject to HHMI’s
Open Access to Publications policy. HHMI lab heads have previously granted a non-exclusive CC BY 4.0 licence to the public and a sublicensable licence to HHMI in their research articles.
Pursuant to those licences, the author-accepted manuscript of this Article can be made freely available under a CC BY 4.0 licence immediately upon publication. AUTHOR INFORMATION Author
notes * Chunxia Zhang Present address: State Key Laboratory of Molecular Developmental Biology, Institute of Genetics and Developmental Biology, Chinese Academy of Sciences, Beijing, China *
These authors contributed equally: Chengjie Zhou, Meng Wang, Chunxia Zhang. AUTHORS AND AFFILIATIONS * Howard Hughes Medical Institute, Boston Children’s Hospital, Boston, MA, USA Chengjie
Zhou, Meng Wang, Chunxia Zhang & Yi Zhang * Program in Cellular and Molecular Medicine, Boston Children’s Hospital, Boston, MA, USA Chengjie Zhou, Meng Wang, Chunxia Zhang & Yi Zhang
* Division of Hematology/Oncology, Department of Pediatrics, Boston Children’s Hospital, Boston, MA, USA Yi Zhang * Department of Genetics, Harvard Medical School, Boston, MA, USA Yi Zhang
* Harvard Stem Cell Institute, Boston, MA, USA Yi Zhang Authors * Chengjie Zhou View author publications You can also search for this author inPubMed Google Scholar * Meng Wang View author
publications You can also search for this author inPubMed Google Scholar * Chunxia Zhang View author publications You can also search for this author inPubMed Google Scholar * Yi Zhang View
author publications You can also search for this author inPubMed Google Scholar CONTRIBUTIONS Y.Z. conceived and supervised the project. C. Zhou and M.W. designed the experiments. C. Zhou
performed the experiments including embryo manipulation, RNA-seq, CUT&RUN, ATAC–seq and so on. M.W. performed all the bioinformatic analysis. C. Zhang established the GABPA-dTAG mice and
GABPA-dTAG ES cells and performed western blot verification. C. Zhou, M.W. and Y.Z. wrote the manuscript. All authors interpreted the data and reviewed the manuscript. CORRESPONDING AUTHOR
Correspondence to Yi Zhang. ETHICS DECLARATIONS COMPETING INTERESTS The authors declare no competing interests. PEER REVIEW PEER REVIEW INFORMATION _Nature Cell Biology_ thanks Sarah Hainer,
Graziano Martello and the other, anonymous, reviewer(s) for their contribution to the peer review of this work. ADDITIONAL INFORMATION PUBLISHER’S NOTE Springer Nature remains neutral with
regard to jurisdictional claims in published maps and institutional affiliations. EXTENDED DATA EXTENDED DATA FIG. 1 GENERATION OF _GABPA_DTAG/DTAG MICE. (A) Diagram of _Fkbp_(F36V)-HA
knock-in at the 3’ end of _Gabpa_ gene. (B) Genotypes of pups from heterozygous _Gabpa_dTAG/+× _Gabpa_dTAG/+ crosses. (C) Confirmation of GABPA degradation with 4h-dTAG13 treatment in zygote
(left) and 4-cell (middle), and anti-HA fluorescence intensity quantification (right) (n = 3). Anti-HA antibody was used for GABPA-HA fusion protein staining. DNA, Hoechst 33342. Scale bar,
20 μm. (D) Quantification of the fluorescence intensity of GABPA in Fig. 1g (n = 3). (E) Embryo development of wild-type embryos with or without dTAG 13 treatment. The developmental rate
(left) and representative pictures of E3.5 embryos (middle and right). Scale bar, 50 μm. The independent experiments were repeated three times. Data in c d and e are presented as mean values
± SD. P-values were calculated with two-sided Student’s t-test. EXTENDED DATA FIG. 2 GENES AFFECTED BY GABPA DEGRADATION AT LATE 2-CELL. (A) Correlations of the replicates of the RNA-seq at
late 2-cell stage. Rp: Pearson correlation. (B) Gene Ontology (GO) terms enriched for all the down-regulated genes after dTAG13 treatment at late 2-cell stage. P-values were adjusted with
Benjamini-Hochberg method. (C) Gene set enrichment analysis (GSEA) of all the down-regulated genes at late 2-cell stage after dTAG13 treatment for ribosome biogenesis (left), mitochondrion
organization (middle) and apoptotic signaling pathway (right). EXTENDED DATA FIG. 3 GABPA BINDING PROFILES AT THE LATE 2-CELL STAGE. (A) Genome browser view showing examples of GABPA
CUT&RUN profiles in ESCs using 500 (n = 1 independent biological replicate) and 20k cells (n = 2 independent biological replicates). (B) Correlation of replicates for GABPA CUT&RUN
with ESCs (top) and late 2-cell embryos (bottom). (C) Heatmaps showing the GABPA binding profiles at promoter, intron and distal regions in late 2-cell embryos. TFs motif occurrence, ATAC
and H3K4me3 signals are shown at the corresponding regions. C: peak center. (D) Heatmaps showing GABPA promoter binding at late 2-cell stage and the corresponding genes expression level with
or without the dTAG13 treatment. (E) Boxplot comparing the expression levels of GABPA targeted genes in DMSO and dTAG-13 treated late 2-cell (For late 2C genes with GABPA promoter binding,
n = 1,991). P-values were calculated with two-sided Mann-Whitney U test. In the boxplot, the central band represents the median. The lower and upper edges of the box represent the first and
third quartiles, respectively. The whiskers of the boxplot extend to 1.5 times interquartile range (IQR). GABPA CUT&RUN analysis in C and D were performed using two independent
biological replicates. ATAC-seq and H3K4me3 CUT&RUN in C showed the pooled results from two biological replicates. EXTENDED DATA FIG. 4 RNA-SEQ IN MORULA AND E4.5 ICM IN RESPONSE TO
DTAG13 TREATMENT AFTER MAJOR ZGA. (A) Diagram showing dTAG13 treatment time window and the time of sample collection for RNA-seq. (B) Correlation of RNA-seq replicates at morula stage. Rp:
Pearson correlation. (C) Dot plot showing the DEGs in morula after dTAG13 treatment shown in panel a. (D) Diagram showing the times of dTAG13 treatment and sample collection. EB: early
blastocyst, MB: middle blastocyst. (E) Immunostaining of NANOG (green) and GATA4 (yellow) for middle blastocyst in control (DMSO) and GABPA degradation (dTAG13) conditions. Scale bar, 20 μm.
(F) Percentage of EPI and PrE cells of middle blastocyst in control (DMSO, n = 22) and GABPA degradation (dTAG13, n = 21) conditions. n indicates the total embryo numbers used in three
independent experiments. P-values were calculated with two-sided Student’s t-test. Data are presented as mean values ± SD. (G) Correlation of replicates of RNA-seq in E4.5 ICM. (H) Volcano
plot showing the DEGs after GABPA degradation in E4.5 ICM. P-values were adjusted with Benjamini-Hochberg method. (I) Boxplot comparing the expression levels of early ICM genes (n = 564),
late ICM genes (n = 243) and early TE genes (n = 698), late TE genes (n = 324) at E4.5 ICM with or without dTAG13 treatment. P-values were calculated with two-sided Mann-Whitney U test. In
the boxplot, the central band represents the median. The lower and upper edges of the box represent the first and third quartiles, respectively. The whiskers of the boxplot extend to 1.5
times interquartile range (IQR). (J) EPI genes and PrE genes identified from single-cell RNA-seq data from Mohammed et al. 99 cells from 10 E3.5 ICM and 105 cells from 5 E4.5 ICM were used
for analysis. (K) Boxplot comparing the expression levels of EPI genes (n = 190) and PrE genes (n = 252) at E4.5 ICM with or without dTAG13 treatment. P-values were calculated with two-sided
Mann-Whitney U test. In the boxplot, the central band represents the median. The lower and upper edges of the box represent the first and third quartiles, respectively. The whiskers of the
boxplot extend to 1.5 times interquartile range (IQR). EXTENDED DATA FIG. 5 GABPA CUT&RUN IN E4.5 ICM. (A) Correlation of replicates for GABPA CUT&RUN in E4.5 ICM. Rp: Pearson
correlation. (B) Heatmaps showing the GABPA binding profiles at promoter, intron and distal regions in E4.5 ICM, and the TFs motif occurrence at the corresponding regions (n = 2 independent
biological replicates). C: peak center. (C) Genome browser view of examples of GABPA binding, ATAC and H3K27ac signals at E4.5 ICM. The binding sites are shaded. (D) Correlation of
replicates for ATAC-seq and H3K27ac CUT&RUN with or without dTAG13 treatment in E4.5 ICM. Rp: Pearson correlation. (E) Heatmap showing GABPA binding (n = 2 independent biological
replicates), ATAC-seq and H3K27ac signals at gene promoters in E4.5 ICM. The genes were separated into two groups based on whether they have promoter GABPA binding. For ATAC and H3K27ac, two
replicates in each condition were merged. GABPA CUT&RUN in C, ATAC-seq and H3K27ac CUT&RUN in C and E showed the pooled results from two biological replicates. EXTENDED DATA FIG. 6
GABPA DEGRADATION IN ESCS. (A) Diagram illustrating the GABPA degradation by dTAG13 treatment in _Gabpa_dTAG/dTAG ESCs. (B) Immunostaining confirming GABPA degradation after dTAG13 treatment
in ESCs (n = 3). Scale bar, 20 μm. Data are presented as mean values ± SD. (C) Correlations of replicates RNA-seq in ESCs. Rp: Pearson correlation. (D) Dot plot showing the DEGs in ESCs
after GABPA degradation. (E) Genomic distribution of GABPA binding peaks in ESCs. (F) Correlation of replicates for ATAC-seq with or without dTAG13 treatment in ESCs. Rp: Pearson
correlation. (G) Heatmaps showing the GABPA binding profiles (n = 2 independent biological replicates) at promoter, intron and distal regions in ESCs, and the TFs motif occurrence and ATAC
signals (Pooled results from two independent biological replicates were showed) at the corresponding regions. C: peak center. (H) Percentage of down-regulated EPI and PrE genes after dTAG13
treatment in ESCs. (I) Comparisons of the down-regulated early and late ICM genes upon dTAG13 treatment in ESCs and E4.5 ICM. EXTENDED DATA FIG. 7 GABPA BINDING DYNAMICS AND COMPARISONS WITH
OTHER PLURIPOTENCY REGULATORS. (A) Expression dynamics of _Gabpa_, _Nr5a2_, _Tfap2c_ and _Sox2_ during mouse pre-implantation development. (B) Correlation of replicates for GABPA
CUT&RUN in 8-cell embryos (left) and E3.5 ICM (right). Rp: Pearson correlation. (C) Genomic distribution of GABPA binding peaks in 8-cell embryos (left) and E3.5 ICM (right). (D) Genome
browser view showing examples of the binding profiles of GABPA in 2-cell, 8-cell, E3.5 ICM, E4.5 ICM and ESCs. (E) Comparison of the GABPA direct target genes between 2-cell embryos and E4.5
ICM. (F) Examples of genome browser view illustrating the GABPA binding (shaded) dynamics during preimplantation development. (G) Heatmap comparison of GABPA and TFAP2C binding profiles at
promoter, intron and distal regions in 2-cell, 8-cell and E3.5 ICM. (H) Comparisons of GABPA (E4.5 ICM) and TFAP2C (E3.5 ICM) affected ICM genes (left) and EPI genes (right). (I) Comparisons
of TFAP2C affected ICM genes (left) and EPI genes (right) in 8-cell and E3.5 ICM. (J) Comparisons of GABPA (E4.5 ICM) and SOX2 (E3.5 ICM) affected ICM genes (left) and EPI genes (right).
(K) Comparisons of GABPA and SOX2 affected ICM genes (left) and EPI genes (right) in ESCs. GABPA CUT&RUN and RNA-seq in E–G showed the pooled results from two biological replicates.
SUPPLEMENTARY INFORMATION REPORTING SUMMARY SUPPLEMENTARY TABLE 1 Gene lists used in this study. SUPPLEMENTARY TABLE 2 DEGs of late two-cell stage treated with GABPA dTAG13 versus that
treated with DMSO. SUPPLEMENTARY TABLE 3 DEGs of morula treated with GABPA dTAG13 versus that treated with DMSO. SUPPLEMENTARY TABLE 4 DEGs of E4.5 ICM treated with GABPA dTAG13 versus that
treated with DMSO. SUPPLEMENTARY TABLE 5 DEGs of ES cell treated with GABPA dTAG13 versus that treated with DMSO. SUPPLEMENTARY TABLE 6 Oligos used in this study. SUPPLEMENTARY TABLE 7 ddPCR
validate GabpadTAG knock-in copy number. SUPPLEMENTARY TABLE 8 Summary of the sequenced libraries in this study. SOURCE DATA SOURCE DATA FIG. 1 Unprocessed western blots for Fig.1. SOURCE
DATA FIG. 2 Statistical source data for Figs. 1–6 and Extended Data Figs. 1–7. RIGHTS AND PERMISSIONS OPEN ACCESS This article is licensed under a Creative Commons Attribution 4.0
International License, which permits use, sharing, adaptation, distribution and reproduction in any medium or format, as long as you give appropriate credit to the original author(s) and the
source, provide a link to the Creative Commons licence, and indicate if changes were made. The images or other third party material in this article are included in the article’s Creative
Commons licence, unless indicated otherwise in a credit line to the material. If material is not included in the article’s Creative Commons licence and your intended use is not permitted by
statutory regulation or exceeds the permitted use, you will need to obtain permission directly from the copyright holder. To view a copy of this licence, visit
http://creativecommons.org/licenses/by/4.0/. Reprints and permissions ABOUT THIS ARTICLE CITE THIS ARTICLE Zhou, C., Wang, M., Zhang, C. _et al._ The transcription factor GABPA is a master
regulator of naive pluripotency. _Nat Cell Biol_ 27, 48–58 (2025). https://doi.org/10.1038/s41556-024-01554-0 Download citation * Received: 20 May 2024 * Accepted: 04 October 2024 *
Published: 02 January 2025 * Issue Date: January 2025 * DOI: https://doi.org/10.1038/s41556-024-01554-0 SHARE THIS ARTICLE Anyone you share the following link with will be able to read this
content: Get shareable link Sorry, a shareable link is not currently available for this article. Copy to clipboard Provided by the Springer Nature SharedIt content-sharing initiative