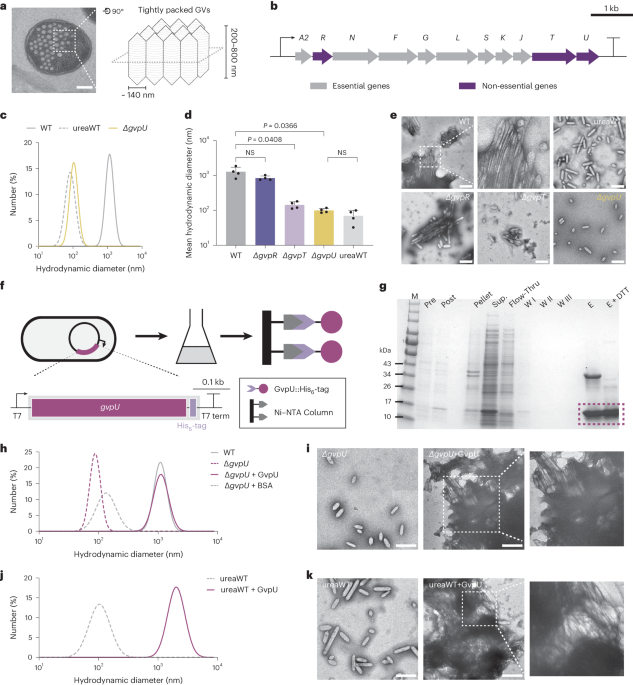
Phase transition of gvpu regulates gas vesicle clustering in bacteria
- Select a language for the TTS:
- UK English Female
- UK English Male
- US English Female
- US English Male
- Australian Female
- Australian Male
- Language selected: (auto detect) - EN
Play all audios:

ABSTRACT Gas vesicles (GVs) are microbial protein organelles that support cellular buoyancy. GV engineering has multiple applications, including reporter gene imaging, acoustic control and
payload delivery. GVs often cluster into a honeycomb pattern to minimize occupancy of the cytosol. The underlying molecular mechanism and the influence on cellular physiology remain unknown.
Using genetic, biochemical and imaging approaches, here we identify GvpU from _Priestia megaterium_ as a protein that regulates GV clustering in vitro and upon expression in _Escherichia
coli_. GvpU binds to the C-terminal tail of the core GV shell protein and undergoes a phase transition to form clusters in subsaturated solution. These properties of GvpU tune GV clustering
and directly modulate bacterial fitness. GV variants can be designed with controllable sensitivity to GvpU-mediated clustering, enabling design of genetically tunable biosensors. Our
findings elucidate the molecular mechanisms and functional roles of GV clustering, enabling its programmability for biomedical applications. Access through your institution Buy or subscribe
This is a preview of subscription content, access via your institution ACCESS OPTIONS Access through your institution Access Nature and 54 other Nature Portfolio journals Get Nature+, our
best-value online-access subscription $29.99 / 30 days cancel any time Learn more Subscribe to this journal Receive 12 digital issues and online access to articles $119.00 per year only
$9.92 per issue Learn more Buy this article * Purchase on SpringerLink * Instant access to full article PDF Buy now Prices may be subject to local taxes which are calculated during checkout
ADDITIONAL ACCESS OPTIONS: * Log in * Learn about institutional subscriptions * Read our FAQs * Contact customer support SIMILAR CONTENT BEING VIEWED BY OTHERS A CATALOG OF THE DIVERSITY AND
UBIQUITY OF BACTERIAL MICROCOMPARTMENTS Article Open access 21 June 2021 ACTIVE PARTICLES INDUCE LARGE SHAPE DEFORMATIONS IN GIANT LIPID VESICLES Article 30 September 2020 COMPOSITION AND
FUNCTIONS OF BACTERIAL MEMBRANE VESICLES Article 17 March 2023 DATA AVAILABILITY The supporting data for findings of this study are available as source data. Models of docking are available
at https://github.com/lab-of-george-lu/Li_2024_NMICROBIOL67. Raw data of disorder profile of GvpU and structure modelling of GvpU are available at
https://github.com/holehouse-lab/supportingdata/tree/master/2024/Li_202468. Macromolecular structural data are available from the RCSB Protein Data Bank (PDB). GvpAAna: PDB 8GBS. GvpA2Mega:
PDB 7R1C. Source data are provided with this paper. CODE AVAILABILITY The computational script used for generating disorder profile of GvpU is available at
https://github.com/holehouse-lab/supportingdata/tree/master/2024/Li_2024. REFERENCES * Greening, C. & Lithgow, T. Formation and function of bacterial organelles. _Nat. Rev. Microbiol._
18, 677–689 (2020). Article CAS PubMed Google Scholar * Uebe, R. & Schüler, D. Magnetosome biogenesis in magnetotactic bacteria. _Nat. Rev. Microbiol._ 14, 621–637 (2016). Article
CAS PubMed Google Scholar * Mauriello, E. Carboxysomes: how bacteria arrange their organelles. _Elife_ 8, e43777 (2019). Article PubMed PubMed Central Google Scholar * Komeili, A.,
Li, Z., Newman, D. K. & Jensen, G. J. Magnetosomes are cell membrane invaginations organized by the actin-like protein MamK. _Science_ 311, 242–245 (2006). Article CAS PubMed Google
Scholar * Scheffel, A. et al. An acidic protein aligns magnetosomes along a filamentous structure in magnetotactic bacteria. _Nature_ 440, 110–114 (2005). Article PubMed Google Scholar *
Savage, D. F., Afonso, B., Chen, A. H. & Silver, P. A. Spatially ordered dynamics of the bacterial carbon fixation machinery. _Science_ 327, 1258–1261 (2010). Article CAS PubMed
Google Scholar * Pfeifer, F. Distribution, formation and regulation of gas vesicles. _Nat. Rev. Microbiol._ 10, 705–715 (2012). Article CAS PubMed Google Scholar * Walsby, A. E. Gas
vesicles. _Microbiol. Rev._ 58, 94–144 (1994). Article CAS PubMed PubMed Central Google Scholar * Bourdeau, R. W. et al. Acoustic reporter genes for non-invasive imaging of
microorganisms in mammalian hosts. _Nature_ 553, 86–90 (2018). Article CAS PubMed PubMed Central Google Scholar * Farhadi, A., Ho, G. H., Sawyer, D. P., Bourdeau, R. W. & Shapiro,
M. G. Ultrasound imaging of gene expression in mammalian cells. _Science_ 365, 1469–1475 (2019). Article CAS PubMed PubMed Central Google Scholar * Sawyer, D. P. et al. Ultrasensitive
ultrasound imaging of gene expression with signal unmixing. _Nat. Methods_ 18, 945–952 (2021). Article CAS PubMed PubMed Central Google Scholar * Lu, G. J. et al. Acoustically modulated
magnetic resonance imaging of gas-filled protein nanostructures. _Nat. Mater._ 17, 456–463 (2018). Article CAS PubMed PubMed Central Google Scholar * Shapiro, M. G. et al. Biogenic gas
nanostructures as ultrasonic molecular reporters. _Nat. Nanotechnol._ 9, 311–316 (2014). Article CAS PubMed PubMed Central Google Scholar * Shapiro, M. G. et al. Genetically encoded
reporters for hyperpolarized xenon magnetic resonance imaging. _Nat. Chem._ 6, 629–634 (2014). Article CAS PubMed Google Scholar * Lu, G. J. et al. Genetically encodable contrast agents
for optical coherence tomography. _ACS Nano_ 14, 7823–7831 (2020). Article CAS PubMed PubMed Central Google Scholar * Hurt, R. C. et al. Genomically mined acoustic reporter genes for
real-time in vivo monitoring of tumors and tumor-homing bacteria. _Nat. Biotechnol._ 41, 919–931 (2023). Article CAS PubMed PubMed Central Google Scholar * Lakshmanan, A. et al.
Acoustic biosensors for ultrasound imaging of enzyme activity. _Nat. Chem. Biol._ 16, 988–996 (2020). Article CAS PubMed PubMed Central Google Scholar * Kim, W. S. et al.
Magneto-acoustic protein nanostructures for non-invasive imaging of tissue mechanics in vivo. _Nat. Mater._ 23, 290–300 (2023). Article PubMed PubMed Central Google Scholar * Bar-Zion,
A. et al. Acoustically triggered mechanotherapy using genetically encoded gas vesicles. _Nat. Nanotechnol._ 16, 1403–1412 (2021). Article CAS PubMed Google Scholar * Wu, D. et al.
Biomolecular actuators for genetically selective acoustic manipulation of cells. _Sci. Adv._ 9, eadd9186 (2023). Article CAS PubMed PubMed Central Google Scholar * Yang, Y. et al.
In-vivo programmable acoustic manipulation of genetically engineered bacteria. _Nat. Commun._ 14, 3297 (2023). Article CAS PubMed PubMed Central Google Scholar * Shen, Q. et al. 50-nm
gas-filled protein nanostructures to enable the access of lymphatic cells by ultrasound technologies. Preprint at _bioRxiv_ https://doi.org/10.1101/2023.06.27.546433 (2023). * Ling, B. et
al. Truly tiny acoustic biomolecules for ultrasound imaging and therapy. _Adv. Mater_ https://doi.org/10.1002/adma.202307106 (2024). * Xie, L., Wang, J., Song, L., Jiang, T. & Yan, F.
Cell-cycle dependent nuclear gene delivery enhances the effects of E-cadherin against tumor invasion and metastasis. _Signal Transduct. Target. Ther._ 8, 182 (2023). Article CAS PubMed
PubMed Central Google Scholar * Song, L. et al. Biogenic nanobubbles for effective oxygen delivery and enhanced photodynamic therapy of cancer. _Acta Biomater._ 108, 313–325 (2020).
Article CAS PubMed Google Scholar * Fernando, A. & Gariépy, J. Coupling chlorin e6 to the surface of nanoscale gas vesicles strongly enhance their intracellular delivery and
photodynamic killing of cancer cells. _Sci. Rep._ 10, 2802 (2020). Article CAS PubMed PubMed Central Google Scholar * Hao, Y., Li, Z., Luo, J., Li, L. & Yan, F. Ultrasound molecular
imaging of epithelial mesenchymal transition for evaluating tumor metastatic potential via targeted biosynthetic gas vesicles. _Small_ 19, e2207940 (2023). Article PubMed Google Scholar
* Anthis, A. H. C. et al. Modular stimuli-responsive hydrogel sealants for early gastrointestinal leak detection and containment. _Nat. Commun._ 13, 7311 (2022). Article CAS PubMed PubMed
Central Google Scholar * Li, N. & Cannon, M. C. Gas vesicle genes identified in _Bacillus megaterium_ and functional expression in _Escherichia coli_. _J. Bacteriol._ 180, 2450–2458
(1998). Article CAS PubMed PubMed Central Google Scholar * Huber, S. T., Terwiel, D., Evers, W. H., Maresca, D. & Jakobi, A. J. Cryo-EM structure of gas vesicles for
buoyancy-controlled motility. _Cell_ 186, 975–986 e913 (2023). Article CAS PubMed PubMed Central Google Scholar * Lakshmanan, A. et al. Preparation of biogenic gas vesicle
nanostructures for use as contrast agents for ultrasound and MRI. _Nat. Protoc._ 12, 2050–2080 (2017). Article CAS PubMed PubMed Central Google Scholar * Offner, S., Hofacker, A.,
Wanner, G. & Pfeifer, F. Eight of fourteen gvp genes are sufficient for formation of gas vesicles in halophilic archaea. _J. Bacteriol._ 182, 4328–4336 (2000). Article CAS PubMed
PubMed Central Google Scholar * Dzuricky, M., Rogers, B. A., Shahid, A., Cremer, P. S. & Chilkoti, A. De novo engineering of intracellular condensates using artificial disordered
proteins. _Nat. Chem._ 12, 814–825 (2020). Article CAS PubMed PubMed Central Google Scholar * Molliex, A. et al. Phase separation by low complexity domains promotes stress granule
assembly and drives pathological fibrillization. _Cell_ 163, 123–133 (2015). Article CAS PubMed PubMed Central Google Scholar * Bremer, A. et al. Deciphering how naturally occurring
sequence features impact the phase behaviours of disordered prion-like domains. _Nat. Chem._ 14, 196–207 (2022). Article CAS PubMed Google Scholar * Shin, Y. & Brangwynne, C. P.
Liquid phase condensation in cell physiology and disease. _Science_ 357, eaaf4382 (2017). Article PubMed Google Scholar * Quiroz, F. G. & Chilkoti, A. Sequence heuristics to encode
phase behaviour in intrinsically disordered protein polymers. _Nat. Mater._ 14, 1164–1171 (2015). Article CAS PubMed PubMed Central Google Scholar * Ruff, K. M., Roberts, S., Chilkoti,
A. & Pappu, R. V. Advances in understanding stimulus-responsive phase behavior of intrinsically disordered protein polymers. _J. Mol. Biol._ 430, 4619–4635 (2018). Article CAS PubMed
Google Scholar * Milo, R. What is the total number of protein molecules per cell volume? A call to rethink some published values. _Bioessays_ 35, 1050–1055 (2013). Article CAS PubMed
PubMed Central Google Scholar * Kar, M. et al. Phase-separating RNA-binding proteins form heterogeneous distributions of clusters in subsaturated solutions. _Proc. Natl Acad. Sci. USA_
119, e2202222119 (2022). Article CAS PubMed PubMed Central Google Scholar * Krainer, G. et al. Reentrant liquid condensate phase of proteins is stabilized by hydrophobic and non-ionic
interactions. _Nat. Commun._ 12, 1085 (2021). Article CAS PubMed PubMed Central Google Scholar * Nott, T. J. et al. Phase transition of a disordered nuage protein generates
environmentally responsive membraneless organelles. _Mol. Cell_ 57, 936–947 (2015). Article CAS PubMed PubMed Central Google Scholar * Bouchard, J. J. et al. Cancer mutations of the
tumor suppressor SPOP disrupt the formation of active, phase-separated compartments. _Mol. Cell_ 72, 19–36 e18 (2018). Article CAS PubMed PubMed Central Google Scholar * Franzmann, T.
M. et al. Phase separation of a yeast prion protein promotes cellular fitness. _Science_ 359, eaao5654 (2018). Article PubMed Google Scholar * Atkinson, J. T. et al. Real-time
bioelectronic sensing of environmental contaminants. _Nature_ 611, 548–553 (2022). Article CAS PubMed Google Scholar * Herud-Sikimić, O. et al. A biosensor for the direct visualization
of auxin. _Nature_ 592, 768–772 (2021). Article PubMed PubMed Central Google Scholar * Quijano-Rubio, A. et al. De novo design of modular and tunable protein biosensors. _Nature_ 591,
482–487 (2021). Article CAS PubMed PubMed Central Google Scholar * Jin, Z. et al. Ultrasonic reporters of calcium for deep tissue imaging of cellular signals. Preprint at _bioRxiv_
https://doi.org/10.1101/2023.11.09.566364 (2023). * Dutka, P. et al. Structure of _Anabaena flos-aquae_ gas vesicles revealed by cryo-ET. _Structure_ 31, 518–528 e516 (2023). Article CAS
PubMed PubMed Central Google Scholar * Banani, S. F., Lee, H. O., Hyman, A. A. & Rosen, M. K. Biomolecular condensates: organizers of cellular biochemistry. _Nat. Rev. Mol. Cell
Biol._ 18, 285–298 (2017). Article CAS PubMed PubMed Central Google Scholar * Dai, Y. et al. Programmable synthetic biomolecular condensates for cellular control. _Nat. Chem. Biol._
119, 518–528 (2023). Article Google Scholar * Mittag, T. & Pappu, R. V. A conceptual framework for understanding phase separation and addressing open questions and challenges. _Mol.
Cell_ 82, 2201–2214 (2022). Article CAS PubMed PubMed Central Google Scholar * Yao, Y. X., Jin, Z. Y., Ling, B., Malounda, D. & Shapiro, M. G. Self-assembly of protein
superstructures by physical interactions under cytoplasm-like conditions. _Biophys. J._ 120, 2701–2709 (2021). Article CAS PubMed PubMed Central Google Scholar * Perez, J. M.,
Josephson, L., O’Loughlin, T., Hogemann, D. & Weissleder, R. Magnetic relaxation switches capable of sensing molecular interactions. _Nat. Biotechnol._ 20, 816–820 (2002). Article CAS
PubMed Google Scholar * Bender, B. J. et al. Protocols for molecular modeling with Rosetta3 and RosettaScripts. _Biochemistry_ 55, 4748–4763 (2016). Article CAS PubMed Google Scholar *
Kozakov, D. et al. The ClusPro web server for protein–protein docking. _Nat. Protoc._ 12, 255–278 (2017). Article CAS PubMed PubMed Central Google Scholar * Desta, I. T., Porter, K.
A., Xia, B., Kozakov, D. & Vajda, S. Performance and its limits in rigid body protein–protein docking. _Structure_ 28, 1071–1081.e1073 (2020). Article CAS PubMed PubMed Central
Google Scholar * Vajda, S. et al. New additions to the ClusPro server motivated by CAPRI. _Proteins Struct. Funct. Bioinforma._ 85, 435–444 (2017). Article CAS Google Scholar * Kozakov,
D. et al. How good is automated protein docking? _Proteins Struct. Funct. Bioinforma._ 81, 2159–2166 (2013). Article CAS Google Scholar * Strunk, T. et al. Structural model of the gas
vesicle protein GvpA and analysis of GvpA mutants in vivo. _Mol. Microbiol._ 81, 56–68 (2011). Article CAS PubMed Google Scholar * Ezzeldin, H. M., Klauda, J. B. & Solares, S. D.
Modeling of the major gas vesicle protein, GvpA: from protein sequence to vesicle wall structure. _J. Struct. Biol._ 179, 18–28 (2012). Article CAS PubMed Google Scholar * Mirdita, M. et
al. ColabFold: making protein folding accessible to all. _Nat. Methods_ 19, 679–682 (2022). Article CAS PubMed PubMed Central Google Scholar * Jumper, J. et al. Highly accurate protein
structure prediction with AlphaFold. _Nature_ 596, 583–589 (2021). Article CAS PubMed PubMed Central Google Scholar * Sievers, F. & Higgins, D. G. Clustal Omega for making accurate
alignments of many protein sequences. _Protein Sci._ 27, 135–145 (2018). Article CAS PubMed Google Scholar * Zakeri, B. et al. Peptide tag forming a rapid covalent bond to a protein,
through engineering a bacterial adhesin. _Proc. Natl Acad. Sci. USA_ 109, E690–E697 (2012). Article CAS PubMed PubMed Central Google Scholar * Milkovic, N. M. & Mittag, T.
Determination of protein phase diagrams by centrifugation. _Methods Mol. Biol._ 2141, 685–702 (2020). Article CAS PubMed Google Scholar * Lu, G. J. lab-of-george-lu/Li_2024_NMICROBIOL.
_GitHub_ https://github.com/lab-of-george-lu/Li_2024_NMICROBIOL (2024). * Holehouse, A. S. holehouse-lab/supportingdata. _GitHub_
https://github.com/holehouse-lab/supportingdata/tree/master/2024/Li_2024 (2024). * Del Conte, A. et al. CAID prediction portal: a comprehensive service for predicting intrinsic disorder and
binding regions in proteins. _Nucleic Acids Res._ 51, W62–W69 (2023). Article PubMed PubMed Central Google Scholar * Tunyasuvunakool, K. et al. Highly accurate protein structure
prediction for the human proteome. _Nature_ 596, 590–596 (2021). Article CAS PubMed PubMed Central Google Scholar * Joshi, V., Shivach, T., Yadav, N. & Rathore, A. S. Circular
dichroism spectroscopy as a tool for monitoring aggregation in monoclonal antibody therapeutics. _Anal. Chem._ 86, 11606–11613 (2014). Article CAS PubMed Google Scholar * Zhang, H. et
al. In situ monitoring of molecular aggregation using circular dichroism. _Nat. Commun._ 9, 4961 (2018). Article PubMed PubMed Central Google Scholar * Grishina, I. B. & Woody, R. W.
Contributions of tryptophan side chains to the circular dichroism of globular proteins: exciton couplets and coupled oscillators. _Faraday Discuss._ 99, 245–262 (1994). Article CAS Google
Scholar * Woody, R. W. Circular dichroism. _Methods Enzymol._ 246, 34–71 (1995). Article CAS PubMed Google Scholar Download references ACKNOWLEDGEMENTS We thank the Shared Equipment
Authority at Rice University for the access to core facilities and instruments and W. Guo for the training. We thank the Thyer Lab at Rice University for providing template plasmids encoding
the spectinomycin resistance gene and the p15A and CloDF13 origins of replication. This work was supported by the Cancer Prevention and Research Institute of Texas, the National Institutes
of Health (R00 EB024600 and R21 EB033607), the Welch Foundation, G. Harold and Leila Y. Mathers Foundation, Hearing Health Foundation and John S. Dunn Foundation. M.I. acknowledges support
from German Research Foundation (DFG) Postdoctoral Fellowship; E.T.U. is supported by a W.M. Keck Fellowship, and Z.L. acknowledges support from Edgar O’Rear and Mary F.D. Morse Travel Award
from the Institute of Biosciences and Bioengineering at Rice University. This work was supported by the Air Force Office of Scientific Research (FA9550-20-1-0241 to L.Y. and A.C). AUTHOR
INFORMATION AUTHORS AND AFFILIATIONS * Department of Bioengineering, Rice University, Houston, TX, USA Zongru Li, Qionghua Shen, Andrew P. Anderson, Manuel Iburg, Richard Lin, Brandon Zimmer
& George J. Lu * Department of Biomedical Engineering, Center for Biomolecular Condensates, Washington University in St. Louis, Saint Louis, MO, USA Emery T. Usher, Alex S. Holehouse
& Yifan Dai * Department of Biochemistry and Molecular Biophysics, Washington University in St. Louis, Saint Louis, MO, USA Emery T. Usher & Alex S. Holehouse * Shared Equipment
Authority, Rice University, Houston, TX, USA Matthew D. Meyer * Department of Biomedical Engineering, Duke University, Durham, NC, USA Lingchong You, Ashutosh Chilkoti & Yifan Dai *
Center for Quantitative BioDesign, Duke University, Durham, NC, USA Lingchong You Authors * Zongru Li View author publications You can also search for this author inPubMed Google Scholar *
Qionghua Shen View author publications You can also search for this author inPubMed Google Scholar * Emery T. Usher View author publications You can also search for this author inPubMed
Google Scholar * Andrew P. Anderson View author publications You can also search for this author inPubMed Google Scholar * Manuel Iburg View author publications You can also search for this
author inPubMed Google Scholar * Richard Lin View author publications You can also search for this author inPubMed Google Scholar * Brandon Zimmer View author publications You can also
search for this author inPubMed Google Scholar * Matthew D. Meyer View author publications You can also search for this author inPubMed Google Scholar * Alex S. Holehouse View author
publications You can also search for this author inPubMed Google Scholar * Lingchong You View author publications You can also search for this author inPubMed Google Scholar * Ashutosh
Chilkoti View author publications You can also search for this author inPubMed Google Scholar * Yifan Dai View author publications You can also search for this author inPubMed Google Scholar
* George J. Lu View author publications You can also search for this author inPubMed Google Scholar CONTRIBUTIONS Conceptualization, G.J.L., A.C., L.Y., Z.L., A.S.H and Y.D.; methodology,
G.J.L., A.C., L.Y., Z.L., Q.S., E.T.U., A.S.H. and Y.D.; investigation, Z.L., Q.S., Y.D., A.S.H., E.T.U., A.P.A., M.I., R.L., B.Z. and M.D.M.; formal analysis, Z.L., Q.S., Y.D., A.S.H.,
E.T.U., A.P.A., M.I., R.L., B.Z. and M.D.M.; writing—original draft, G.J.L., A.C., L.Y., Z.L., Q.S., A.S.H. and Y.D.; supervision and funding acquisition, G.J.L., Y.D., A.C. and L.Y.
CORRESPONDING AUTHORS Correspondence to Lingchong You, Ashutosh Chilkoti, Yifan Dai or George J. Lu. ETHICS DECLARATIONS COMPETING INTERESTS Z.L., Q.S, and G.J.L. are co-inventors on a US
provisional patent application that incorporates discoveries described in this paper. Their interests are reviewed and managed by Rice University in accordance with their conflict of
interest policies. The other authors declare no competing interests. PEER REVIEW PEER REVIEW INFORMATION _Nature Microbiology_ thanks Jon Marles-Wright and the other, anonymous, reviewer(s)
for their contribution to the peer review of this work. ADDITIONAL INFORMATION PUBLISHER’S NOTE Springer Nature remains neutral with regard to jurisdictional claims in published maps and
institutional affiliations. EXTENDED DATA EXTENDED DATA FIG. 1 MASS SPECTRUM OF GVPU::HIS6-TAG OBTAINED FROM MALDI TOF MS. The x-axis displays the mass-to-charge ratio (m/z) of the ionized
species, while the Y-axis represents the ion intensity. The presence of the peak with 15681.311 m/z in the spectrum confirms the identity of GvpU::His6-tag. Source data EXTENDED DATA FIG. 2
ALIGNMENT OF CRYO-EM STRUCTURES OF MAJOR SHELL PROTEIN GVPAANA AND GVPA2MEGA. GvpAAna is depicted in yellow and GvpA2Mega in grey. The secondary structures, adapted from cryo-EM structures
for GvpAAna (PDB: 8GBS)49 and GvpA2Mega (PDB: 7R1C)30, are segmented into the N-terminal (Nt) and C-terminal (Ct) regions, two α-helices (α1 and α2), and two β-strands (β1 and β2). Notably,
both cryo-EM structures did not resolve the C-terminal tail due to its disordered conformations, and we labeled the last residue visible from the cryo-EM structures on the figure. EXTENDED
DATA FIG. 3 PHASE DIAGRAM OF PURIFIED GVPU PROTEINS, WHICH SHOWS A TYPICAL UCST PHASE TRANSITION BEHAVIOR. Source data EXTENDED DATA FIG. 4 MCHERRY DOES NOT FORM TIME-DEPENDENT PUNCTA IN _E.
COLI_. _E. coli_ induced with 0.05 mM IPTG grown for a) 5 and b) 8 hours from inoculation (2 and 5 hours after induction). Scale bar = 5 µm. EXTENDED DATA FIG. 5 GVPU IS PREDICTED TO
ASSEMBLE INTO DEFINED HOMO-OLIGOMERS OF 5-7 UNITS. A) The average overall pLDDT70 score for all chains in different oligomeric states. In general, regardless of oligomeric state, the
monomeric protein fold is predicted with relatively high confidence and is essentially identical, although trimer, tetramer, and octamers show lower pLDDT than pentamer, hexamer, and
heptamers. The data are boxplots of the pLDDT scores for all residues in the various oligomeric complexes; thus, there is one pLDDT score for each residue in the complex. n = 138 (monomer),
276 (dimer), 414 (trimer), 552 (tetramer), 690 (pentamer), 828 (hexamer), 966 (heptamer), and 1104 (octamer). The minima is the lower adjacent value; the maxima is the upper adjacent value;
the box is drawn from the 25th and 75th percentiles with the median as the horizontal line in center. Whiskers are truncated at min/max of data if they would overstep the data. B) The
predicted 3D structure obtained from AlphaFold2 for monomeric GvpU. The structure is colored according to the pLDDT score (blue = more confident, red = less confident). C–I) Predicted 3D
structure of dimers-thru-octamers and the Predicted Alignment Error (PAE). These data do not enable us to confidently assess which of pentamer, hexamer, and heptamer is most likely, although
naively, the pentamer is the most confident overall prediction in terms of pLDDT and PAE. All models predict the same overall topology regardless of assembly start with a short (8-residue)
hydrophobic low-confidence N-terminal region extending upwards (with respect to the right-hand-side representation) and a second short (8-residue) positively-charged low-confidence
C-terminal region extending downwards. Both these regions are predicted to be disordered (Fig. 4a). In both cases, these short extensions are positioned adjacent to the equivalent region
from the next protomer. Taken together, our structural model suggests these short disordered regions form a meshwork on the top and bottom of the oligomer. EXTENDED DATA FIG. 6
CONCENTRATION-DEPENDENT ASSEMBLY OF GVPU YIELDS ANOMALOUS SPECTROSCOPIC FEATURES. A) Far-UV circular dichroism (CD) spectra of three concentrations of GvpU. At the lowest concentration (1.27
μM = 0.02 mg/mL, lightest purple) of GvpU, noise below 220 nm precludes certainty in secondary structure assignment. Qualitatively, the local minimum at ~222 nm (denoted by vertical dashed
line) may reflect some α-helical character at 1.27 μM. Notably, the spectra for the two higher concentrations do not trend as expected for a system of non-interacting chromophores71,72.
Presented as molar ellipticity73,74, which is normalized to sample concentration and size, the spectra differ in shape and intensity. Specifically, as GvpU concentration increases, the
minima of the spectra decrease in negative intensity and undergo a modest red shift (higher wavelength), despite a linear increase in absorbance signal with increasing concentration. B)
Considering evidence from dynamic light scattering for the concentration-dependent formation of soluble oligomers of GvpU, the emergent CD spectral features at 12.7 and 22.9 μM likely
reflect interactions between chromophores in separate GvpU subunits. In particular, the AF2 model for the GvpU homomeric assembly implicates hydrophobic packing at the subunit interface;
drawing from exciton coupling theory, we speculate that the interactions between Phe and Tyr at the hydrophobic interface could be responsible for the observed hypochromic shift in the CD
signal at concentrations that support self-assembly. Source data EXTENDED DATA FIG. 7 REPRESENTATIVE TEM IMAGES OF GV VARIANTS. A) wildtype GvpA2Mega -based GVs (WT), B) WT GVs with _gvpU_
middle region Phe to Ser mutation (F → S), C) _gvpU_ middle region Glu to Ser/Gly mutations (E → S, G), D) and _gvpU_ middle region Phe/Glu to Ser/Gly mutations (F, E → S, G). GV clustering
in WT and E → S, G variants are zoomed in. Scale bar = 500 nm. Source data EXTENDED DATA FIG. 8 INTER-SUBUNIT INTERFACE OF THE PREDICTED GVPU PENTAMER. The predicted structure of the
pentameric oligomer with the hydrophobic interface between two protomers highlighted. A network of aromatic and aliphatic residues (with one threonine) line the interior of the interface,
with F71 and F57 forming a central pair of residues to connect the two protomers via hydrophobic interactions with the adjacent F26, L28, and F17 of the next protomers. EXTENDED DATA FIG. 9
GVPC DOES NOT INTERFERE WITH GVPU-MEDIATED GV CLUSTERING. A) Representative DLS measurement of A2Mega,2C_∆gvpU_ constructs (n = 3 for biologically independent samples). B) Representative TEM
images of A2Mega,2C_∆gvpU_ constructs (scale bar, 500 nm). Source data SUPPLEMENTARY INFORMATION SUPPLEMENTARY INFORMATION Supplementary Table 1 and Methods (sequences of oligonucleotides
and reagent or kit catalogue number). REPORTING SUMMARY SUPPLEMENTARY TABLE 1 Sequences of oligonucleotides and reagent or kit catalogue number. SOURCE DATA SOURCE DATA FIG. 1 Statistical
source data for Fig. 1c,d,h,j. SOURCE DATA FIG. 1 Unprocessed SDS–PAGE gel. SOURCE DATA FIG. 1 Unprocessed TEM micrographs. SOURCE DATA FIG. 2 Statistical source data for Fig. 2c,d,g,j,l.
SOURCE DATA FIG. 2 Unprocessed SDS–PAGE gel and western blot. SOURCE DATA FIG. 2 Unprocessed TEM micrographs. SOURCE DATA FIG. 3 Statistical source data for Fig. 3d,e,f,g,h,i. SOURCE DATA
FIG. 3 Unprocessed micrographs. SOURCE DATA FIG. 4 Statistical source data for Fig. 4d,f. SOURCE DATA FIG. 4 Unprocessed TEM micrographs. SOURCE DATA FIG. 5 Statistical source data for Fig.
5d,e,g,i,k. SOURCE DATA FIG. 5 Unprocessed SDS–PAGE gel (labelled). SOURCE DATA FIG. 5 Unprocessed TEM micrographs. SOURCE DATA EXTENDED DATA FIG. 1 Statistical source data for Extended Data
Fig. 1. SOURCE DATA EXTENDED DATA FIG. 3 Statistical source data for Extended Data Fig. 3. SOURCE DATA EXTENDED DATA FIG. 6 Statistical source data for Extended Data Fig. 6. SOURCE DATA
EXTENDED DATA FIG. 7 Unprocessed TEM micrographs. SOURCE DATA EXTENDED DATA FIG. 9 Statistical source data for Extended Data Fig. 9a. SOURCE DATA EXTENDED DATA FIG. 9 Unprocessed TEM
micrograph. RIGHTS AND PERMISSIONS Springer Nature or its licensor (e.g. a society or other partner) holds exclusive rights to this article under a publishing agreement with the author(s) or
other rightsholder(s); author self-archiving of the accepted manuscript version of this article is solely governed by the terms of such publishing agreement and applicable law. Reprints and
permissions ABOUT THIS ARTICLE CITE THIS ARTICLE Li, Z., Shen, Q., Usher, E.T. _et al._ Phase transition of GvpU regulates gas vesicle clustering in bacteria. _Nat Microbiol_ 9, 1021–1035
(2024). https://doi.org/10.1038/s41564-024-01648-3 Download citation * Received: 02 July 2023 * Accepted: 20 February 2024 * Published: 29 March 2024 * Issue Date: April 2024 * DOI:
https://doi.org/10.1038/s41564-024-01648-3 SHARE THIS ARTICLE Anyone you share the following link with will be able to read this content: Get shareable link Sorry, a shareable link is not
currently available for this article. Copy to clipboard Provided by the Springer Nature SharedIt content-sharing initiative