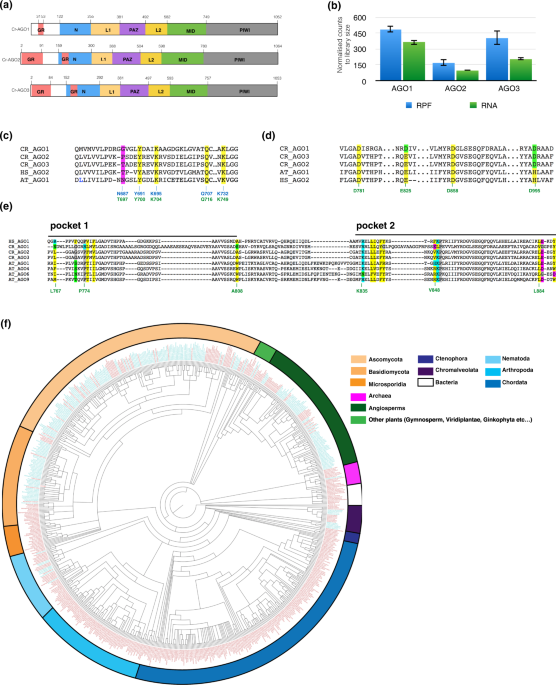
Distinct roles of argonaute in the green alga chlamydomonas reveal evolutionary conserved mode of mirna-mediated gene expression
- Select a language for the TTS:
- UK English Female
- UK English Male
- US English Female
- US English Male
- Australian Female
- Australian Male
- Language selected: (auto detect) - EN
Play all audios:

ABSTRACT The unicellular green alga _Chlamydomonas reinhardtii_ is evolutionarily divergent from higher plants, but has a fully functional silencing machinery including microRNA
(miRNA)-mediated translation repression and mRNA turnover. However, distinct from the metazoan machinery, repression of gene expression is primarily associated with target sites within
coding sequences instead of 3′UTRs. This feature indicates that the miRNA-Argonaute (AGO) machinery is ancient and the primary function is for post transcriptional gene repression and
intermediate between the mechanisms in the rest of the plant and animal kingdoms. Here, we characterize AGO2 and 3 in _Chlamydomonas_, and show that cytoplasmically enriched Cr-AGO3 is
responsible for endogenous miRNA-mediated gene repression. Under steady state, mid-log phase conditions, Cr-AGO3 binds predominantly miR-C89, which we previously identified as the
predominant miRNA with effects on both translation repression and mRNA turnover. In contrast, the paralogue Cr-AGO2 is nuclear enriched and exclusively binds to 21-nt siRNAs. Further
analysis of the highly similar Cr-AGO2 and Cr-AGO 3 sequences (90% amino acid identity) revealed a glycine-arginine rich N-terminal extension of ~100 amino acids that, given previous work on
unicellular protists, may associate AGO with the translation machinery. Phylogenetic analysis revealed that this glycine-arginine rich N-terminal extension is present outside the animal
kingdom and is highly conserved, consistent with our previous proposal that miRNA-mediated CDS-targeting operates in this green alga. SIMILAR CONTENT BEING VIEWED BY OTHERS RNA-GUIDED RNA
SILENCING BY AN ASGARD ARCHAEAL ARGONAUTE Article Open access 29 June 2024 DIPEPTIDYL PEPTIDASE DPF-3 IS A GATEKEEPER OF MICRORNA ARGONAUTE COMPENSATION IN ANIMALS Article Open access 20
March 2025 LINKING KEY STEPS OF MICRORNA BIOGENESIS BY TREX-2 AND THE NUCLEAR PORE COMPLEX IN _ARABIDOPSIS_ Article 20 July 2020 INTRODUCTION The diverse natural roles for RNA-silencing in
eukaryotes range from defense against viruses to the regulation of gene expression and chromatin remodeling1,2,3,4,5,6,7,8,9. The central player of this pathway is the evolutionarily
conserved ribonuclease protein – Argonaute (AGO). Sequence specificity of the RNA-silencing pathway is facilitated by 20–30 nucleotide (nt) small RNAs (sRNAs) that bind to the AGO protein
and guide it to RNAs with complementary target sites, leading to silencing of the target messengers via translational repression and/or degradation. AGO proteins are present almost
throughout the eukaryote kingdom, thus suggesting that the RNA silencing pathway is ancient and was present in the last eukaryotic common ancestor, where it most likely functioned in defense
against viruses and transposons10. AGO proteins are also present in prokaryotes but are associated with DNA instead of RNA11,12. Eukaryote AGO proteins are part of the PIWI-protein
superfamily, defined by the presence of a PIWI (P element-induced wimpy testes) domain12. They have been divided into four major clades – the Trypanosoma AGO clade, the WAGO (worm Argonaute)
clade, the AGO-like clade and the PIWI-like clade12. AGO-like proteins are present in plants, fungi and animals, whereas PIWI-like are specific to the germline in animals. Four domains are
conserved in eukaryotic AGOs: the N-domain, which plays a critical role in target cleavage and in the dissociation of strands after cleavage13,14,15,16; the PAZ domain, which binds to the 3′
end of the associated small RNA17,18; the MID domain which contains a basic binding pocket that recognizes the 5′ phosphate of the associated small RNA19 and may discriminate between the
various 5′ end nucleotides20,21; and the PIWI domain, which contains the RNase H-like active site and the catalytic triad DDX (where X is usually aspartic acid or histidine or occasionally
lysine)22,23. The haploid unicellular green alga _Chlamydomonas reinhardtii_ has a complex set of small RNAs and diverse RNA silencing pathways ranging from miRNA-mediated cleavage of target
mRNA to translational repression24,25,26,27,28,29. The _Chlamydomonas_ genome contains three _AGO_ genes with _Cr-AGO1_ being more divergent than _Cr-AGO2_ and _3_. AGO1 has a likely role
in genome defense against transposable elements30, while Cr-AGO3 is probably responsible for miRNA-mediated transcript cleavage and translational repression31. In this work, we further
characterize _Chlamydomonas_ AGO proteins in order to understand the evolution of this protein family in eukaryotes. We show that Cr-AGO2 is preferentially nuclear localized, while Cr-AGO3
is cytoplasmic enriched and associates with polysomes. IP experiments confirmed that Cr-AGO3 associates with miRNAs, while Cr-AGO2 exclusively binds siRNAs. The most prevalent miRNA bound to
Cr-AGO3 is miR-C89, the only known endogenous miRNA that mediates both translation repression and mRNA turnover1. We also further characterize the structure of the three AGO proteins in
_Chlamydomonas_ revealing differences in length of an unstructured extension of the N-domain, enriched in glycine and arginine residues (GR-N). We extend the analysis of this domain to all
annotated AGO proteins, revealing that while this domain is generally absent in AGO proteins from archaea, bacteria, microsporidia and animals, it is conserved in almost all other
eukaryotes. Based on these findings we propose that the GR-N domain might facilitate coding region targeting by AGO proteins as demonstrated in our previous study1. RESULTS AND DISCUSSION
COMPUTATIONAL ANALYSIS OF _CHLAMYDOMONAS_ AGO DOMAIN STRUCTURE The _Chlamydomonas reinhardtii_ genome encodes three AGOs - Cr-AGO1 (109 kDa), Cr-AGO2 (109 kDa) and Cr-AGO3 (108 kDa)
[phytozome V10.1]. Similar to other eukaryotic AGOs, each protein contains all conserved domains (in order of N to C terminus) - N, PAZ, MID and PIWI, where the PAZ domain is linked to the N
and MID domains by two linker regions, linker 1 and 2 (L1 and L2), respectively (Fig. 1a, Supplementary Fig. 1a). From the ribosome profiling data in our previous study1, which is
essentially a direct measurement of protein synthesis, it appears that Cr-AGO1 is the most abundant of the three AGO proteins, followed by Cr-AGO3 then Cr-AGO2, which is in agreement with
RNA abundance (Fig. 1b). In order to characterize Cr-AGOs and identify structural differences that might indicate functional specialization, we estimated Cr-AGO structures by threading the
amino acid sequences through the crystalized human AGO2 structure32. We focused on three localized signatures that characterize specific AGO functions: (1) the MID-domain residues known to
interact with the 5′ phosphate residue for small RNAs and can be responsible for small RNA sorting; (2) the catalytic tetrad in the PIWI domain required for target cleavage; and (3) the
tryptophan binding pockets utilised for heterodimer formation with tryptophan-containing proteins. All three Cr-AGOs possess the highly conserved 5′ phosphate binding residues in the MID
domain33 - corresponding to K127, Y123, Q159 and K163 in _A_. _fulgidus_ AGO34 or Y691, K695, Q707 and K732 in _A_. _thaliana_ AGO1 which also contributes to its 5′ nt selectivity20. This
finding suggests that all three Cr-AGOs may interact with small RNAs containing a 5′ phosphate group. The corresponding key residue affecting binding of the 5′ residue of the sRNA (N687 in
At-AGO1) differs between Cr-AGO2 and 3 which suggested that, similar to At-AGOs, Cr-AGO2 and 3 have the capacity for differential sRNA binding specificity (Fig. 1c and Supplementary Fig.
1b). Further, analysis of Hs-AGO2 demonstrates that I365 on helix 7 that promotes the targeting rule based on seed-pairing35. Both helix 7 and residue I365 are highly conserved in Cr-AGO2
and 3 (Supplementary Fig. 1d) consistent with a similar targeting mode between animal and _Chlamydomonas_ proposed following our previous global analysis1. As for potential slicer activity,
both Cr-AGO2 and 3 possess identical catalytic tetrad residues (DEDD) required for slicer activity in the PIWI domain as At-AGO1 and HS-AGO2 (Fig. 1d)33,36. The conservation of these
residues implies a potential ability for slicing activity for Cr-AGO2 and 3 as previously suggested37. However, the slicing activity could also be dependent on coordination with the
N-terminal domain, as described for metazoan AGOs13,14,15,38. In Cr-AGO1, the corresponding tetrad residues are DDDD, instead of DEDD. As the difference is a conservative change between two
negatively charged residues (glutamic acid to aspartic acid), it is likely that Cr-AGO1 also has potential slicing activity. Cr-AGO2 and 3, but not Cr-AGO1 possess the hydrophobic pockets
required for interaction with tryptophan-containing proteins such as GW-182 and silencing suppressors such as P38 of Turnip crinkle virus39 (Fig. 1e and Supplementary Fig. 1c). This feature
suggests both Cr-AGO2 and 3 have the potential to recruit tryptophan-containing partners to modulate their silencing activities. GLYCINE-ARGININE (GR)-ENRICHED REGIONS IN CR-AGOS AND THEIR
PREVALENCE IN OTHER EUKARYOTIC AGO PROTEINS The sequences of all three AGOs have a predicted unstructured N-terminal extension of the N-domain. This domain is enriched in glycine and
arginine (GR) residues that are involved in protein-RNA interactions due to the positively charged arginine residues40,41. Cr-AGO1 has a shorter GR-rich stretch of 22 amino acids (aa), while
in Cr-AGO2 and Cr-AGO3 this region spans more than half the N-terminal extension of the canonical N-domain. In addition, another GR-rich stretch of ~20 amino acid is found within the
canonical N-terminal domain of both Cr-AGO2 and Cr-AGO3 (Fig. 1a and Supplementary Fig. 1a). It is likely Cr-AGO2 and 3 have similar functions due to their high degree of homology (89% aa
identity to each other)37,42 while Cr-AGO1 is distinct from Cr-AGO2 and Cr-AGO3 (35% aa identity to both Cr-AGO2 and Cr-AGO3). Based on the domains defined by threading, the non-conservative
differences between Cr-AGO2 and Cr-AGO3 are within the unstructured GR-N, the canonical N-terminal domain and the MID domain. Most differences within the GR-N are either insertion/deletions
of runs of glycine (see Supplementary Fig. 1e for both conservative and non-conservative differences and Supplementary Fig. 1f for alignment of Cr-AGO2 and Cr-AGO3). Our recent work using
_Ago3_ and _Dcl3_ mutants demonstrated that endogenous _C_. _reinhartdii_ miRNA primarily represses endogenous gene expression by targeting coding regions (CDSs) instead of 3′UTRs as in
metazoan systems1. Therefore, we hypothesize that this CDS- specific process is dependent on an interaction of Cr-AGO3 with the ribosome via the GR-N extension40,43,44. The loss of the
interaction between the polysome and trypanosome AGO1 by mutagenesis of the GR-N3 supports this model. It is possible that other AGO proteins with GR-N extension in higher plants, protozoa,
and most fungi may also target coding sequence motifs in mRNAs. To investigate the evolutionary prevalence of GR-rich N-terminal (GR-N) domains in AGOs, all 7026 AGO protein sequences from
548 species currently available in NCBI were scanned for GR-N extension throughout the whole protein sequence. For the purposes of this analysis, we defined GR-N extensions based on the
occurrence of at least one contiguous region of length between 8 and 100 aa containing a minimum of 50% glycine and/or alanine residues and a minimum of 30% arginine residues. With these
parameters, 991 sequences (in 252 species) were found to contain GR-N sequence. Surprisingly, most of the 991 GR-N-containing AGOs are from fungi, _Chromalveolata_ and plant kingdoms, and
the GR-N extensions are upstream of the N-domain (Fig. 1f and Supplementary Fig. 1g). Within the animal kingdom, a long GR-N sequence was only detected within the nematode phylum. Many
species have multiple isoforms of AGO including those either with or without the GR-N extension. For example, only AGOs that associate with 21nt miRNA in _Arabidopsis thaliana_ – namely 1,
2, 3 and 5 have a GR-N extension that is >60 aa. The AGOs associated with 24-nt small RNAs, namely AGO4, 6 and 9 (Supplementary Fig. 1h) do not have these extension. The near
exclusiveness of GR-N extensions to three kingdoms - fungi (except the division of Microspordia), plant and Chromalveolata - indicates that this domain is eukaryotic-specific and may have
been lost during evolution of AGO proteins in complex Metazoans. There are, however, exceptional animal PIWI-like proteins with GR-N motifs45 in which some of the arginines are methylated
and are docking sites for the binding of various germline Tudor proteins45,46,47. The GR-N motif of Cr-AGO1 is reminiscent these PIWI GR-N motifs that are generally much shorter than the
motifs in AGO proteins (Supplementary Fig. 1i,j). EXPRESSION AND LOCALIZATION OF THE CR-AGO PROTEINS Based on our computational analysis, we propose that Cr-AGO2 and/or 3 rather than Cr-AGO1
are the likely candidates for miRNA and siRNA-mediated gene expression. To further characterize the Cr-AGO proteins responsible for miRNA- or siRNA-mediated gene expression, we raised
antibodies against peptides from the PAZ and PIWI domains for both Cr-AGO2 and 3 (Fig. 2a), and used them for both western blot and immunoprecipitation. Due to similarity between Cr-AGO2 and
3, the antibodies raised were not able to differentiate between these proteins. Therefore we utilized a loss of function AGO3 mutant, _ago3-25_ to specifically detect AGO2 protein and
compare with the wild type strain, where antibodies recognized both AGO2 and AGO3 proteins. The mutant _ago3-25_ was obtained in a forward genetic screen (Supplementary Fig. 2)26 and does
not accumulate AGO3 mRNA1. Both anti-PAZ and PIWI antibodies recognized a prominent band in the wild type (parental) and a less abundant band in the _ago_3-25 mutant (Fig. 2b, Supplementary
Fig. 2c). In order to confirm the specificity of our antibodies, we immunoprecipitated parental and _ago3-25_ mutant protein extracts with the anti-PAZ antibody. The precipitate was detected
by the anti-PIWI antibody, and LC-MS/MS analysis confirmed the presence of both Cr-AGO2 and 3 in the parental strain, and Cr-AGO2 and not AGO3 in the _ago3-25_ mutant (Supplementary Fig. 2
d–f). Therefore, both antibodies can specifically detect endogenous levels of Cr-AGO2 and Cr-AGO3. To distinguish the role between Cr-AGO2 and 3, we next investigated their cellular
localizations because AGO proteins in plants and animals may be either nuclear or cytoplasmic48. Cytoplasmic AGOs, such as At-AGO1, are normally involved in miRNA-mediated gene repression
(translational repression or enhanced RNA turnover). To investigate localization of Cr-AGOs 2/3, we performed western blots from cytoplasmic- and nuclear-enriched extracts from both the
parental (P) and _ago3-25_ (M) strains. As expected, histone H3 was enriched in the nuclear fraction (N), while depleted from the cytoplasmic fraction (C) (Fig. 2c). The signal corresponding
to Cr-AGO-2/3 proteins was detected in the nuclear fraction of the parental strain and _ago3-25_ mutant, indicating that AGO2 protein is predominantly nuclear. In contrast, we detected
AGO-2/3 signal in the cytoplasmic fractions of the parental strain only. We conclude, therefore, that this signal corresponds to Cr-AGO3 (Fig. 2c, Supplementary Fig. 2g) and that Cr-AGO3 is
predominantly cytoplasmic while Cr-AGO2 is mostly nuclear. We found further support for AGO3 cytoplasmic localization by assaying the presence of Cr-AGO proteins in purified polysomes.
Cr-AGO-2/3 was detected in both total and polysomal fractions of parental extracts; however, this signal was less abundant from the polysomal fraction of _ago3-25_ lines (Supplementary Fig.
2h). There is however a very faint band in sample _ago3-25-_polysome-C which is overall more concentrated than all other polysome samples, possibly a reflection of residual AGO2 in cytoplasm
interacting with the ribosome through its GR-N domain. The cytoplasmic, polysome-associated localization of Cr-AGO3 is therefore consistent with its role in post-transcriptional
regulation1,37. CR-AGO2 AND CR-AGO3 REQUIRE PRESENCE OF DCL3 FOR STABILITY The high degree of similarity between Cr-AGO2 and 3 suggests they are associated with similar species of small RNA
despite being differentially localized. To test if both Cr-AGO2 and 3 are associated with 21-nt small RNAs, we investigated their stability in a DCL3 knockout background, _dcl3-1_, in which
production of 21-nt siRNA and miRNA is greatly reduced1,26. Figure 3a shows Cr-AGO-2/3protein abundance detected by the PAZ antibody in the _dcl3-1_ mutant and in its corresponding
complemented line. Similar to many reports demonstrating requirement of small RNA-association for stability in metazoan AGOs49,50,51, Cr- AGO-2/3 was significantly reduced in the _dcl3-1_
mutant, suggesting that the reduction of 21-nt sRNAs prevents the accumulation of Cr-AGO2/3 proteins (Fig. 3a and Supplementary Fig. 2i). The reduction of Cr-AGO2/3 protein levels could be a
consequence of a decrease in its mRNA levels or translation, or an increase in protein turnover. To differentiate these possibilities, we analyzed previous ribosome profiling and RNA-seq
data1, which showed no significant change in the RNA abundance and translation of both Cr-AGO2 and AGO3 in the _dcl3-1_ mutant, suggesting that the reduced level of these proteins in _dcl3_
mutant is a consequence of a higher protein turnover (Fig. 3b and Supplementary Fig. 2j). Therefore, these results indicate that, similarly to metazoan AGO proteins, Cr-AGO2 and/or Cr-AGO3
are stabilized by association with small RNAs49,50,51. CR-AGO3 BUT NOT CR-AGO2 PRIMARILY ASSOCIATES WITH MIRNA To further explore the possibility that Cr-AGO2 and 3 bind different
populations of sRNA we sequenced the RNA bound to immunoprecipitated Cr-AGO2 + 3/2 from lysates of either the parental or _ago3-25_ strains to allow for comparative Cr-AGO2 and 3 small RNA
analysis (Fig. 2b and Supplementary Fig. 3a). We rationalized that, in the parental background, the IP product would be a mixture of Cr-AGO2 and 3 while, in the _ago3-25_ background, the IP
product would be specifically Cr-AGO2. An Arabidopsis AGO1 antibody (α-At-AGO1) was used as an IP negative control (Fig. 2b). This analysis also included total sRNA libraries from parental
(P) and (M) _ago3-25_ cultures. To reduce sequence bias from PCR amplification and ligation, NEXTflex adaptors containing four random terminal nucleotides were used for preparation of the
small RNA libraries. It is important to utilize adaptors with random terminal nucleotides, also known as HD-tags as it has been established that conventional small RNA library preparation
methods limits accurate quantification of miRNAs due to severe ligation bias1,52,53,54,55,56. The final analysis was based on the sequence reads that mapped to the _Chlamydomonas_ nuclear
genome (Phytozome 281). Furthermore, we also re-defined DCL3-dependent small RNA loci via segmentSeq57, utilizing small RNA libraries generated from both the _dcl3-1_ mutant and its
complement line1,26. The new list of loci was utilized for all siRNA analyses in this study (Supplementary Table 1) and it differs from the earlier version in that all, rather than most,
miRNAs are derived from mRNA precursors58. Figure 4a presents the relative abundance and read-length distribution of total small RNA libraries in _dcl3-1_, complement of _dcl3-1_, _ago3-25_
(M) and complemented (C) and Parental (P) lines for filtered reads, reads that maps to annotated miRNA precursors, DCL3-dependent siRNA loci, and reads that map to the rest of the nuclear
genome but not annotated miRNA precursor nor siRNA loci utilized in this study. The miRNA reads are primarily 21 nt whereas siRNA reads range from 20–22 nt with 21 nt as the primary size
class. Similar to vascular plants, the abundance of siRNA in _C_. _reinhardtii_ is significantly greater than miRNA and the abundance of some siRNAs and miRNAs is dependent on the presence
of AGO3 (Fig. 4a,b). In addition, in correspondence with previously reported small RNA-seq in a different AGO3 mutant background, small RNAs with 5′ terminal uridine are significantly
reduced while the abundance of those with 5′ terminal adenosine increased in the absence of AGO3 (Fig. 4c)31. The reduced concentration of miRNA and siRNA with 5′ U in the absence of Cr-AGO3
was further confirmed by northern blotting (Fig. 4d and Supplementary Fig. 3b,c and Supplementary Table 1)26. The miR-C89 with 5′ A, however, was more abundant in _ago3-25_ than in the
parent, also confirmed by northern blot (Fig. 4d,e, Supplementary Fig. 3b and Supplementary Table 2). These finding suggests that, at least in _Chlamydomonas_, stability of miRNAs with a 5′
terminal uridine is AGO3-dependent. Figure 5a presents corresponding small RNA-Seq data of the IP in Fig. 2b. In order to enrich for the most abundant AGO2/3-associated small RNAs and
minimize background, the IP was performed under high salt conditions ([NaCl] = 0.8 M) that do not impact AGO2/3 enrichment while significantly decreasing background proteins (Supplementary
Fig. 2d). The absence of 21nt miRNA and enrichment of siRNA in _ago3-_25-IP background further supports Cr-AGO3 as the primary miRNA effector and both Cr-AGO3 and 2 are effectors for siRNA.
It is striking that Cr-AGO2 exclusively associates with siRNA with 5′ adenosine and that miR-C89 (also with 5′ adenosine) dominates Cr-AGO3-associated miRNAs (Fig. 5b,c). The predominant
enrichment of miR-C89 further supports our previous observation that this is the most prevalent endogenous miRNA for regulating gene expression under steady-state conditions1. Thus we
conclude that the miR-C89-AGO3 complex is the primary endogenous miRNA complex for post-transcriptional gene regulation in _Chlamydomonas_. It is striking that miR-C89 with a 5′ adenosine
accounts for most of the miRNA bound to Cr-AGO3 corresponding to it being the most abundant miRNA in our sRNA datasets1,26. This finding differs from previous studies showing miRNA bound to
Cr-AGO3 with a 5′ uridine58. The difference is likely due, in part, to (1) our utilization of adaptors with randomized terminal nucleotides to reduce biased ligation to small RNA with 5′
uridine and PCR duplication of reads52 and (2) the stringent IP condition (NaCl = 0.8 M) so that only the most abundant or tightly bound AGO2/3-associated small RNAs would be retained. Our
isolation of small RNA from endogenous instead of over-expressed tagged-AGO ensures capturing of biologically relevant interactions between functional AGO and small RNAs and may also
contribute to the difference in our dataset and that of Voshall _et al_. (2015). miR-C89 is unique amongst the miRNAs in that the precursor structure is embedded within the coding region of
a gene (Cre05.g239950) (Fig. 5d). All other miRNAs are derived from non coding regions of coding mRNAs including introns1,26. Cre05.g239950 is more abundant in the _dcl3-1_ background
(Supplementary Fig. 4 of Chung _et al_. 2017) consistent with its stability being inversely affected by processing of miRNA precursor1. In contrast, however, similar to other
miRNA-containing genes, the level of Cre05.g239950 is not significantly affected by presence nor lack of AGO3 suggesting Cr-AGO3 is unlikely to be involved in miR-C89 processing (Fig. 5e and
Supplementary Fig. 3d). To explain the higher level of miR-C89 in _ago3-25_ background than in its wild type parent we propose that turnover of this miRNA is accelerated when it is
associated with AGO3 (Figs 4d,e and 5e). Further analysis of the contrasting properties of miR-C89 and other miRNAs in association with Cr-AGO3 will be informative about the biology and
molecular biology of RNA silencing in _C_. _reinhardtii_. MATERIALS AND METHODS CULTURE AND SAMPLE PREPARATION Fresh, independent colonies of _Chlamydomonas reinhardtii_ cells (CC-1883) were
cultured and maintained as previously described1. For IP, lysates were prepared by harvesting cells in mid-log phase (OD750~0.6). Cell cultures were incubated on ice for 30 min prior to
centrifugation at 4000 rpm, 5 min, 4 °C. The pellet was re-suspended in IP lysis buffer (1 × PBS, NaF 50 mM, NaSVO4 1 mM, MgCl2 5 mM, PMSF 1 mM, NP40 0.1%, DTT 5 mM, NaCl 0.8 M, 1X protease
inhibitor cocktail without EDTA (Roche)). The resuspended cells were then dounce homogenised on ice 20×, followed by centrifugation at 4700 rpm, 4 °C, 30 min. The clarified samples were
either snap frozen in liquid nitrogen or 10% TCA-acetone precipitated and resuspended in resuspension buffer (Urea 8 M, NaCl 0.5 M and DTT 5 mM) for western blot analysis.
IMMUNOPRECIPITATION AND SMALL RNA LIBRARY PREPARATION Two antibodies α-2/3-PAZ: GVRGGPAQLAAADP and α-2/3-PIWI: PTGAAARADSRDPS (GenScript and Agrisera, AS142799) were raised for all
immuno-detection analyses. All western blots were performed with 1/500 overnight 4 °C incubation with the primary antibody and 1/5000 anti-rabbit for 1 h at room temperature prior to
development. All immunoprecipitations were performed with antibodies conjugated to tosylactivated streptavadin beads M280 (Invitrogen) and immunoprecipitation was performed following the
manufacturer’s protocol after the last wash step, the beads were spilt in half for either directly boiling in SDS-PAGE sample buffer for western blot or total RNA extraction followed by
small RNA library preparation as described previously1. RNA EXTRACTION AND SMALL RNA NORTHERN BLOT RNA was extracted from cell cultures grown to a cell density of 2–3 × 106 cells/ml in TAP
media, at 23 °C and under constant light. RNA extraction was performed as previously described59, with the following modifications: RNA was extracted from live cells after centrifugation of
the culture, without prior freezing; PureZol (Biorad) was used instead of Trizol and volumes were scaled down to perform extraction in 2 ml tubes. RNA quality was assessed in gel and
quantify in Nanodrop (ThermoFisher Scientific). Small RNA detection by Northern blot was performed as described in59
(http://www.plantsci.cam.ac.uk/research/davidbaulcombe/methods/downloads/smallrna.pdf/view). 15 µg of total RNA was separated in 15% TBE-Urea gels (Invitrogen) and transfer by capillarity to
an Amersham Hybond N+ membrane (GE Healthcare). A DNA oligo corresponding to the reverse complementarity sequence of the small RNA to assay was radiolabeled with γ32P-ATP (PerkinElmer)by
the action of the T4 polynucleotide kinase (ThermoFisher Scientific), and used to probe membranes with the immobilized RNA samples. Radioactive signals were detected using a storage phosphor
screen (GE Healthcare), which was scanned using a Typhoon 9400 imager (GE Healthcare). For detection of U6 RNA species, used as a loading control, a fluorescent-labeled DNA oligo was
employed, and blots were scanned using an Odyssey Imaging System (LI-COR). Images were analyzed using the ImageJ software. Oligo sequences used for small RNA northern blot: miR-C89:
5′CATTCCACACTTTTCACGCCT3′, miR1157: 5′CACCTGGTCCCGCTACCTGAA3′ and U6: 5′IRD800/TAACAGGTTATGAGCCCCGG3′ DIFFERENTIAL ISOLATION OF PROTEINS Cells were similarly harvested as for IP lysates
except resuspended cells were subjected to 2× freeze thaw cycle followed by centrifugation at 14000 rpm, 5 min, 4 °C. The Pellet was washed 3× with 1 ml IP lysis buffer containing NP40 0.5%
followed by TCA-acetone precipitation for total nuclear protein extraction. The supernatant was also TCA-acetone precipitated for total cytoplasmic protein extraction. Total polysome protein
was obtained by addition of 100 µg/ml cycloheximide and 100 µg/ml chloramphenicol to 100 ml cells in mid-log phase for 5 min followed by incubation in ice for 10 min and pelleted at 4700
rpm for 1 min at 4 °C. The cell pellet was resuspended in 1 ml ice cold polysome buffer (Tris-Cl pH 7.5 10 mM, KCl 140 mM, MgCl2 5 mM, DTT 0.5 mM, NP40 0.5%, TritonX100 1%, PMSF 1 mM, NaF 50
mM, NaSVO4 1 mM, 1X roche protease inhibitor without EDTA cocktail, 100 µg/ml cycloheximide and 100 µg/ml chloramphenicol and SUPERase Inhibitor 10 U/ml, TurboDNase 5 U/ml). The
resuspension was passed through 30 G needle twice, followed by centrifugation at 14000 rpm, 10 min, 4 °C. The supernatant was layered on 8 ml of 35% sucrose made in polysome buffer and
centrifuged at 38000 rpm, 18 h, 4 °C in a SW41 rotor. The pellet was then TCA-acetone precipitated for western blot analysis. Anti-histone3 antibody (Agrisera #AS15 2855) and Anti-ribosomal
protein S3 (Cell Signalling #2579) were utilised to assess enrichment of nuclear or cytoplasmic extract. MASS SPECTROMETRY OF CR-AGO2 AND AGO3 1D gel bands were transferred into a 96-well
PCR plate. The bands were cut into 1 mm2 pieces, destained, reduced (DTT) and alkylated (iodoacetamide) and subjected to enzymatic digestion with chymotrypsin overnight at 37 °C. After
digestion, the supernatant was pipetted into a sample vial and loaded onto an autosampler for automated LC-MS/MS analysis. All LC-MS/MS experiments were performed using a Dionex Ultimate
3000 RSLC nanoUPLC (Thermo Fisher Scientific Inc, Waltham, MA, USA) system and a Q Exactive Orbitrap mass spectrometer (Thermo Fisher Scientific Inc, Waltham, MA, USA). Separation of
peptides was performed by reverse-phase chromatography at a flow rate of 300 nL/min and a Thermo Scientific reverse-phase nano Easy-spray column (Thermo Scientific PepMap C18, 2 µm particle
size, 100 A pore size, 75 µm i.d. × 50 cm length). Peptides were loaded onto a pre-column (Thermo Scientific PepMap 100 C18, 5 µm particle size, 100 A pore size, 300 µm i.d. × 5 mm length)
from the Ultimate 3000 autosampler with 0.1% formic acid for 3 minutes at a flow rate of 10 µL/min. After this period, the column valve was switched to allow elution of peptides from the
pre-column onto the analytical column. Solvent A was water + 0.1% formic acid and solvent B was 80% acetonitrile, 20% water + 0.1% formic acid. The linear gradient employed was 2–40% B in 30
minutes. The LC eluant was sprayed into the mass spectrometer by means of an Easy-Spray source (Thermo Fisher Scientific Inc.). All _m/z_ values of eluting ions were measured in an Orbitrap
mass analyzer, set at a resolution of 70000 and was scanned between _m/z_ 380–1500. Data dependent scans (Top 20) were employed to automatically isolate and generate fragment ions by higher
energy collisional dissociation (HCD, NCE:25%) in the HCD collision cell and measurement of the resulting fragment ions was performed in the Orbitrap analyser, set at a resolution of 17500.
Singly charged ions and ions with unassigned charge states were excluded from being selected for MS/MS and a dynamic exclusion window of 20 seconds was employed. Post-run, the data was
processed using Protein Discoverer (version 2.1., ThermoFisher). Briefly, all MS/MS data were converted to mgf files and the files were then submitted to the Mascot search algorithm (Matrix
Science, London UK) and searched against a customised NCBI _Chlamydomonas reinhardtii_ database (14491 sequences; 6575547 residues) and a common contaminant sequences (115 sequences, 38274
residues). Variable modifications of oxidation (M), deamidation (NQ) and carbamidomethyl were applied. The peptide and fragment mass tolerances were set to 5 ppm and 0.1 Da, respectively. A
significance threshold value of p < 0.05 and a peptide cut-off score of 20 were also applied. COMPUTATIONAL ANALYSIS To define regions of AGO containing GR-N extension, 7026 currently
available AGO protein sequences were extracted from the refeseq database in NCBI and scanned for GR-N extension using a combination of C-shell C++ scripts. The scanning parameter for GR-N
extension were based on the occurrence of at least one contiguous region of length between 8 and 100 aa containing a minimum of 50% glycine and/or alanine residues and a minimum of 30%
arginine residues. The purpose to include alanine is based on the hypothesis that the key interactor is the positively charged arginine residue which is positioned by small, uncharged
restudies such as glycine and alanine. The output was then post-processed by a combination of C-shell scripts and the statistical package R. The phylogenic tree were produced by FigTree
(v1.4.2)60. Differential expression of mRNA were performed using DE-Seq2 and Small RNA data processing were performed as described in Chung _et al_. 2017. The software package segmentSeq was
utilized for identification and differential expression analysis of 21-nt DCL3-dependent small RNA loci57. The reference genome and transcriptome of _Chlamydomonas reinhardtii_ used were
Phytozome v5.0 and 281, respectively. DATA AVAILABILITY All raw sequencing data are deposited on Arrayexpress with accessions E-MTAB-5726. All code used for bioinformatic analysis is
available on request. REFERENCES * Chung, B. Y.-W., Deery, M. J., Groen, A. J., Howard, J. & Baulcombe, D. C. Endogenous miRNA in the green alga Chlamydomonas regulates gene expression
through CDS-targeting. _Nat. Plants_ 3, 787–794 (2017). Article CAS Google Scholar * Braun, L. _et al_. A complex small rna repertoire is generated by a plant/fungal-like machinery and
effected by a metazoan-like argonaute in the single-cell human parasite toxoplasma gondii. _PLoS Pathog._ 6, 1–20 (2010). Article Google Scholar * Shi, H., Ullu, E. & Tschudi, C.
Function of the trypanosome argonaute 1 protein in RNA interference requires the N-terminal RGG domain and arginine 735 in the Piwi domain. _J. Biol. Chem._ 279, 49889–49893 (2004). Article
CAS Google Scholar * Hannon, G. J. & Rossi, J. J. Unlocking the potential of the human genome with RNA interference. _Nature_ 431, 371–378 (2004). Article ADS CAS Google Scholar
* Mello, C. C. & Conte, D. _Revealing the world of RNA interference_. _Nature_ 431 (2004). * Lippman, Z. & Martienssen, R. The role of RNA interference in heterochromatic silencing.
_Nature_ 431, 364–370 (2004). Article ADS CAS Google Scholar * Baulcombe, D. RNA silencing in plants. _Nature_ 431, 356–363 (2004). Article ADS CAS Google Scholar * Meister, G. &
Tuschi, T. Mechanisms of gene silencing by double-stranded RNA. _Nature_ 431, 343–349 (2004). Article ADS CAS Google Scholar * Ambros, V. The functions of animal microRNAs. _Nature_
431, 350–355 (2004). Article ADS CAS Google Scholar * Shabalina, S. A. & Koonin, E. V. Origins and evolution of eukaryotic RNA interference. _Trends in Ecology and Evolution_ 23,
578–587 (2008). Article Google Scholar * Swarts, D. C. _et al_. DNA-guided DNA interference by a prokaryotic Argonaute. _Nature_ 507, 258–61 (2014). Article ADS CAS Google Scholar *
Swarts, D. C. _et al_. The evolutionary journey of Argonaute proteins. _Nat. Struct. Mol. Biol._ 21, 743–53 (2014). Article CAS Google Scholar * Faehnle, C. R., Elkayam, E., Haase, A. D.,
Hannon, G. J. & Joshua-Tor, L. The Making of a Slicer: Activation of Human Argonaute-1. _Cell Rep._ 3, 1901–1909 (2013). Article CAS Google Scholar * Nakanishi, K. _et al_.
Eukaryote-Specific Insertion Elements Control Human ARGONAUTE Slicer Activity. _Cell Rep._ 3, 1893–1900 (2013). Article CAS Google Scholar * Hauptmann, J. _et al_. Turning catalytically
inactive human Argonaute proteins into active slicer enzymes. _Nat. Struct. Mol. Biol._ 20, 814–7 (2013). Article CAS Google Scholar * Kwak, P. B. & Tomari, Y. The N domain of
Argonaute drives duplex unwinding during RISC assembly. _Nat. Struct. Mol. Biol._ 19, 145–51 (2012). Article CAS Google Scholar * Lingel, A., Simon, B., Izaurralde, E. & Sattler, M.
Structure and nucleic-acid binding of the Drosophila Argonaute 2 PAZ domain. _Nature_ 426, 465–9 (2003). Article ADS CAS Google Scholar * Yan, K. S. _et al_. Structure and conserved RNA
binding of the PAZ domain. _Nature_ 426, 468–474 (2003). Article ADS Google Scholar * Wang, Y. _et al_. Structure of an argonaute silencing complex with a seed-containing guide DNA and
target RNA duplex. _Nature_ 456, 921–926 (2008). Article ADS CAS Google Scholar * Frank, F., Hauver, J., Sonenberg, N. & Nagar, B. Arabidopsis Argonaute MID domains use their
nucleotide specificity loop to sort small RNAs. _EMBO J._ 31, 3588–95 (2012). Article CAS Google Scholar * Zha, X., Xia, Q. & Adam Yuan, Y. Structural insights into small RNA sorting
and mRNA target binding by Arabidopsis Argonaute Mid domains. _FEBS Lett._ 586, 3200–3207 (2012). Article CAS Google Scholar * Song, J., Smith, S. K. & Hannon, G. J. Crystal Structure
of Argonaute Slicer Activity. _Science (80-.)._ 305, 1434–1437 (2004). Article ADS CAS Google Scholar * Liu, J. _et al_. Argonaute2 is the catalytic engine of mammalian RNAi. _Science_
305, 1437–41 (2004). Article ADS CAS Google Scholar * Molnar, a, Schwach, F., Studholme, D. J., Thuenemann, E. C. & Baulcombe, D. C. miRNAs control gene expression in the single-cell
alga Chlamydomonas reinhardtii. _Nature_ 447, 1126–1129 (2007). Article ADS CAS Google Scholar * Molnar, A. _et al_. Highly specific gene silencing by artificial microRNAs in the
unicellular alga Chlamydomonas reinhardtii. _Plant J._ 58, 165–74 (2009). Article CAS Google Scholar * Valli, A. A. _et al_. Most microRNAs in the single-cell alga Chlamydomonas
reinhardtii are produced by Dicer-like 3-mediated cleavage of introns and untranslated regions of coding RNAs. _Genome Res._ 26, 519–529 (2016). Article CAS Google Scholar * Yamasaki, T.
_et al_. Complementarity to an miRNA seed region is sufficient to induce moderate repression of a target transcript in the unicellular green alga Chlamydomonas reinhardtii. _Plant J._ 76,
1045–1056 (2013). Article CAS Google Scholar * Shaver, S., Casas-Mollano, J. A., Cerny, R. L. & Cerutti, H. Origin of the polycomb repressive complex 2 and gene silencing by an E(z)
homolog in the unicellular alga Chlamydomonas. _Epigenetics_ 5, 301–12 (2010). Article CAS Google Scholar * Yamasaki, T., Onishi, M., Kim, E.-J., Cerutti, H. & Ohama, T. RNA-binding
protein DUS16 plays an essential role in primary miRNA processing in the unicellular alga _Chlamydomonas reinhardtii_. _Proc_. _Natl_. _Acad_. _Sci_. 201523230,
https://doi.org/10.1073/pnas.1523230113 (2016). Article CAS Google Scholar * Casas-Mollano, J. A. _et al_. Diversification of the core RNA interference machinery in Chlamydomonas
reinhardtii and the role of DCL1 in transposon silencing. _Genetics_ 179, 69–81 (2008). Article CAS Google Scholar * Yamasaki, T., Kim, E. J., Cerutti, H. & Ohama, T. Argonaute3 is a
key player in miRNA-mediated target cleavage and translational repression in Chlamydomonas. _Plant J._ 85, 258–268 (2016). Article CAS Google Scholar * Schirle, N. T., Sheu-Gruttadauria,
J. & MacRae, I. J. Structural basis for microRNA targeting. _Science (80-.)._ 346, 608–613 (2014). Article ADS CAS Google Scholar * Song, J.-J., Smith, S. K., Hannon, G. J. &
Joshua-Tor, L. Crystal structure of Argonaute and its implications for RISC slicer activity. _Science_ 305, 1434–7 (2004). Article ADS CAS Google Scholar * Ma, J. B. _et al_. Structural
basis for 5′-end-specific recognition of guide RNA by the A. fulgidus Piwi protein. _Nature_ 434, 666–670 (2005). Article ADS CAS Google Scholar * Klum, S. M., Chandradoss, S. D.,
Schirle, N. T., Joo, C. & MacRae, I. J. Helix-7 in Argonaute2 shapes the microRNA seed region for rapid target recognition. _EMBO J_. e201796474, https://doi.org/10.15252/embj.201796474
(2017). Article Google Scholar * Cuperus, J. T. _et al_. Functional Analysis of Three Arabidopsis ARGONAUTES Using Slicer-Defective Mutants. _The Plant Cell_ 24, 3613–3629 (2012). Article
Google Scholar * Yamasaki, T., Kim, E.-J., Cerutti, H. & Ohama, T. Argonaute3 is a key player in miRNA-mediated target cleavage and translational repression in Chlamydomonas. _Plant
J._ 85, 258–68 (2016). Article CAS Google Scholar * Hur, J. K., Zinchenko, M. K., Djuranovic, S. & Green, R. Regulation of Argonaute slicer activity by guide RNA 3??? end interactions
with the N-terminal lobe. _J. Biol. Chem._ 288, 7829–7840 (2013). Article CAS Google Scholar * Azevedo, J. _et al_. Argonaute quenching and global changes in Dicer homeostasis caused by
a pathogen-encoded GW repeat protein. _Genes Dev._ 24, 904–15 (2010). Article CAS Google Scholar * Castello, A. _et al_. Comprehensive Identification of RNA-Binding Domains in Human
Cells. _Mol. Cell_ 63, 696–710 (2016). Article CAS Google Scholar * Vasilyev, N. _et al_. Crystal structure reveals specific recognition of a G-quadruplex RNA by a β-turn in the RGG motif
of FMRP. _Proc. Natl. Acad. Sci. USA_ 112, E5391–400 (2015). Article CAS Google Scholar * Cerutti, H. & Casas-Mollano, J. A. On the origin and functions of RNA-mediated silencing:
From protists to man. _Curr. Genet._ 50, 81–99 (2006). Article CAS Google Scholar * Järvelin, A. I., Noerenberg, M., Davis, I. & Castello, A. The new (dis)order in RNA regulation.
_Cell Commun. Signal._ 14, 9 (2016). Article Google Scholar * Thandapani, P., O’Connor, T. R., Bailey, T. L. & Richard, S. Defining the RGG/RG Motif. _Molecular Cell_ 50, 613–623
(2013). Article CAS Google Scholar * Kirino, Y. _et al_. Arginine methylation of Piwi proteins catalysed by dPRMT5 is required for Ago3 and Aub stability. _Nat. Cell Biol._ 11, 652–8
(2009). Article CAS Google Scholar * Chen, C. _et al_. Mouse Piwi interactome identifies binding mechanism of Tdrkh Tudor domain to arginine methylated Miwi. _Proc. Natl. Acad. Sci. USA_
106, 20336–41 (2009). Article ADS CAS Google Scholar * Vagin, V. V. _et al_. Proteomic analysis of murine Piwi proteins reveals a role for arginine methylation in specifying interaction
with Tudor family members. _Genes Dev._ 23, 1749–1762 (2009). Article CAS Google Scholar * Meister, G. Argonaute proteins: functional insights and emerging roles. _Nat. Rev. Genet._ 14,
447–59 (2013). Article CAS Google Scholar * Smibert, P., Yang, J.-S., Azzam, G., Liu, J.-L. & Lai, E. C. Homeostatic control of Argonaute stability by microRNA availability. _Nat.
Struct. Mol. Biol._ 20, 789–95 (2013). Article CAS Google Scholar * Johnston, M., Geoffroy, M.-C., Sobala, A., Hay, R. & Hutvagner, G. HSP90 Protein Stabilizes Unloaded Argonaute
Complexes and Microscopic P-bodies in Human Cells. _Mol. Biol. Cell_ 21, 1462–1469 (2010). Article CAS Google Scholar * Elkayam, E. _et al_. The structure of human argonaute-2 in complex
with miR-20a. _Cell_ 150, 100–110 (2012). Article CAS Google Scholar * Sorefan, K. _et al_. Reducing ligation bias of small RNAs in libraries for next generation sequencing. _Silence_ 3,
4 (2012). Article CAS Google Scholar * Kim, H. _et al_. Bias-minimized quantification of microRNA reveals widespread alternative processing and 3′ end modification. _Nucleic Acids Res._
47, 2630–2640 (2019). Article Google Scholar * Kim, H. _et al_. AQ-seq: Accurate quantification of microRNAs and their variants, https://doi.org/10.1101/339606. * Xu, P. _et al_. An
improved protocol for small RNA library construction using High Definition adapters, https://doi.org/10.1515/mngs-2015-0001 (2015). * Fowler, E. K., Mohorianu, I., Smith, D. T., Dalmay, T.
& Chapman, T. Small RNA populations revealed by blocking rRNA fragments in Drosophila melanogaster reproductive tissues. _PLoS One_ 13, e0191966 (2018). Article Google Scholar *
Hardcastle, T. J., Kelly, K. A. & Baulcombe, D. C. Identifying small interfering RNA loci from high-throughput sequencing data. _Bioinformatics_ 28, 457–63 (2012). Article CAS Google
Scholar * Voshall, A., Kim, E. J., Ma, X., Moriyama, E. N. & Cerutti, H. Identification of AGO3-associated miRNAs and computational prediction of their targets in the green alga
Chlamydomonas reinhardtii. _Genetics_ 200, 105–121 (2015). Article CAS Google Scholar * Molnár, A., Schwach, F., Studholme, D. J., Thuenemann, E. C. & Baulcombe, D. C. miRNAs control
gene expression in the single-cell alga Chlamydomonas reinhardtii. _Nature_ 447, 1126–1129 (2007). Article ADS Google Scholar * Rambaut, A. FigTree v1.3.1. 2006–2009. _Accessed Novemb_.
29, 2012 Program package available at, http://tree.bio.ed.ac (2009). Download references ACKNOWLEDGEMENTS We thank J. Barlow for technical assistance and media preparation; T.J. Hardcastle
for technical bioinformatic support. This work was supported by a Balzan Prize award and the European Research Council Advanced Investigator Grant ERC-2013-AdG 340642 TRIBE. B.Y.W.C. was
supported by an EMBO long-term postdoctoral fellowship, a Sir Henry Wellcome Fellowship [096082] and a Medical Research Council Careers Development Fellowship [MR/R021821/1]. D.C.B. is the
Royal Society Edward Penley Abraham Research Professor. AUTHOR INFORMATION Author notes * Betty Y.-W. Chung Present address: Department of Pathology, University of Cambridge, Cambridge, CB2
1QP, United Kingdom * Adrian Valli Present address: Department of Plant Molecular Genetics, Spanish National Centre for Biotechnology, Madrid, 28049, Spain AUTHORS AND AFFILIATIONS *
Department of Plant Sciences, University of Cambridge, Cambridge, CB2 3EA, United Kingdom Betty Y.-W. Chung, Adrian Valli, Francisco J. Navarro, Silvia Hnatova & David C. Baulcombe *
Department of Pathology, University of Cambridge, Cambridge, CB2 1QP, United Kingdom Katherine Brown * Cambridge System Biology Centre and Department of Biochemistry, University of
Cambridge, Cambridge, CB2 1GA, United Kingdom Michael J. Deery & Julie Howard * Institute of Molecular Plant Sciences, University of Edinburgh, Edinburgh, EH9 3BF, United Kingdom Attila
Molnar Authors * Betty Y.-W. Chung View author publications You can also search for this author inPubMed Google Scholar * Adrian Valli View author publications You can also search for this
author inPubMed Google Scholar * Michael J. Deery View author publications You can also search for this author inPubMed Google Scholar * Francisco J. Navarro View author publications You can
also search for this author inPubMed Google Scholar * Katherine Brown View author publications You can also search for this author inPubMed Google Scholar * Silvia Hnatova View author
publications You can also search for this author inPubMed Google Scholar * Julie Howard View author publications You can also search for this author inPubMed Google Scholar * Attila Molnar
View author publications You can also search for this author inPubMed Google Scholar * David C. Baulcombe View author publications You can also search for this author inPubMed Google Scholar
CONTRIBUTIONS B.Y.W.C. and D.C.B. conceived and designed the research. B.Y.W.C. performed most of the experiments and analysed the data. F.J.N. performed the small RNA northern blots, A.V.
and S.H. mapped the _ago3-25_ mutation. A.V. isolated and characterised the _ago3-25_ mutant and its complement and carried out the northern blots in Supplementary Fig. 3c. K.A.B. performed
mRNA differential analysis. F.J.N. and A.M. commented and revised the manuscript. _M_.J.D. and J.H. performed all the LC-MS/MS sample processing. B.Y.W.C. and D.C.B. wrote the manuscript.
CORRESPONDING AUTHORS Correspondence to Betty Y.-W. Chung or David C. Baulcombe. ETHICS DECLARATIONS COMPETING INTERESTS The authors declare no competing interests. ADDITIONAL INFORMATION
PUBLISHER’S NOTE: Springer Nature remains neutral with regard to jurisdictional claims in published maps and institutional affiliations. SUPPLEMENTARY INFORMATION SUPPLEMENTARY INFORMATION
RIGHTS AND PERMISSIONS OPEN ACCESS This article is licensed under a Creative Commons Attribution 4.0 International License, which permits use, sharing, adaptation, distribution and
reproduction in any medium or format, as long as you give appropriate credit to the original author(s) and the source, provide a link to the Creative Commons license, and indicate if changes
were made. The images or other third party material in this article are included in the article’s Creative Commons license, unless indicated otherwise in a credit line to the material. If
material is not included in the article’s Creative Commons license and your intended use is not permitted by statutory regulation or exceeds the permitted use, you will need to obtain
permission directly from the copyright holder. To view a copy of this license, visit http://creativecommons.org/licenses/by/4.0/. Reprints and permissions ABOUT THIS ARTICLE CITE THIS
ARTICLE Chung, B.YW., Valli, A., Deery, M.J. _et al._ Distinct roles of Argonaute in the green alga _Chlamydomonas_ reveal evolutionary conserved mode of miRNA-mediated gene expression. _Sci
Rep_ 9, 11091 (2019). https://doi.org/10.1038/s41598-019-47415-x Download citation * Received: 17 January 2019 * Accepted: 11 July 2019 * Published: 31 July 2019 * DOI:
https://doi.org/10.1038/s41598-019-47415-x SHARE THIS ARTICLE Anyone you share the following link with will be able to read this content: Get shareable link Sorry, a shareable link is not
currently available for this article. Copy to clipboard Provided by the Springer Nature SharedIt content-sharing initiative