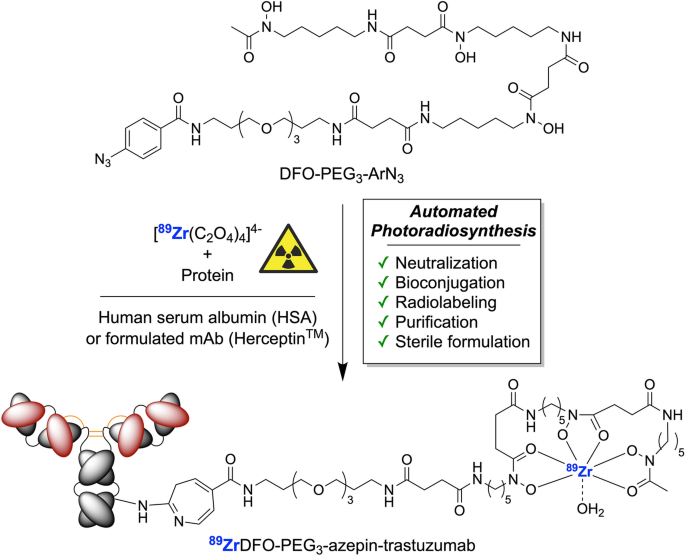
Automated light-induced synthesis of 89zr-radiolabeled antibodies for immuno-positron emission tomography
- Select a language for the TTS:
- UK English Female
- UK English Male
- US English Female
- US English Male
- Australian Female
- Australian Male
- Language selected: (auto detect) - EN
Play all audios:

ABSTRACT Clinical production of 89Zr-radiolabeled antibodies (89Zr-mAbs) for positron emission tomography imaging relies on the pre-conjugation of desferrioxamine B (DFO) to the purified
protein, followed by isolation and characterization of the functionalized intermediate, and then manual radiosynthesis. Although highly successful, this route exposes radiochemists to a
potentially large radiation dose and entails several technological and economic hurdles that limit access of 89Zr-mAbs to just a specialist few Nuclear Medicine facilities worldwide. Here,
we introduce a fully automated synthesis box that can produce individual doses of 89Zr-mAbs formulated in sterile solution in < 25 min starting from [89Zr(C2O4)4]4– (89Zr-oxalate), our
good laboratory practice-compliant photoactivatable desferrioxamine-based chelate (DFO-PEG3-ArN3), and clinical-grade antibodies without the need for pre-purification of protein. The
automated steps include neutralization of the 89Zr-oxalate stock, chelate radiolabeling, and light-induced protein conjugation, followed by 89Zr-mAb purification, formulation, and sterile
filtration. As proof-of-principle, 89ZrDFO-PEG3-azepin-trastuzumab was synthesized directly from Herceptin in < 25 min with an overall decay-corrected radiochemical yield of 20.1 ± 2.4%
(_n_ = 3), a radiochemical purity > 99%, and chemical purity > 99%. The synthesis unit can also produce 89Zr-mAbs via the conventional radiolabeling routes from pre-functionalized
DFO-mAbs that are currently used in the clinic. This automated method will improve access to state-of-the-art 89Zr-mAbs at the many Nuclear Medicine and research institutions that require
automated devices for radiotracer production. SIMILAR CONTENT BEING VIEWED BY OTHERS A SCALABLE PROTOCOL FOR THE RADIOSYNTHESIS OF CLINICAL GRADE LUTETIUM-177-LABELED THERANOSTIC AGENTS
Article 27 May 2025 SYNTHESIS OF 68GA-RADIOPHARMACEUTICALS USING BOTH GENERATOR-DERIVED AND CYCLOTRON-PRODUCED 68GA AS EXEMPLIFIED BY [68GA]GA-PSMA-11 FOR PROSTATE CANCER PET IMAGING Article
04 March 2022 INVERSE ELECTRON DEMAND DIELS–ALDER CLICK CHEMISTRY FOR PRETARGETED PET IMAGING AND RADIOIMMUNOTHERAPY Article 14 June 2021 INTRODUCTION Automation is common practice in the
synthesis of clinical-grade radiopharmaceuticals. For example, the small-molecule, metal-based radiotracers 68Ga-PSMA-11 and 177Lu-PSMA-617 are typically produced by automated synthesis
modules that perform both the radiolabeling and purification steps1,2,3,4. In these examples, the chemical reaction is a one-step process (metal ion complexation) and efficient radiolabeling
produces the isolated and fully formulated radiopharmaceutical with high decay-corrected radiochemical yields (RCYs > 80%) and radiochemical purity (RCP > 95%). It is important to
note that the metalation step typically runs to full decay-corrected radiochemical conversion (RCC > 95%). Activity losses stem from inefficient transfer along fluid pathways or imperfect
recovery during the purification step. More complex chemical reactions or multiple synthetic steps can also be automated but these processes are usually encountered only in the production
of small-molecule 11C- and 18F-labeled PET tracers5,6,7. For instance, 11C-radiochemistry often requires the use of non-aqueous solvents and the synthesis of reactive intermediates, such as
11C-methyl iodide, before subsequent reactions with a precursor give the desired radiotracer. In 11C- and 18F-radiochemistry, the labeling precursors often contain protected functional
groups that are unmasked by deprotection steps following the introduction of the radionuclide8,9,10,11. This chemical flexibility facilitates the ‘_Total Radiosynthesis_’ of complex drug
molecules where the radionuclide is incorporated in positions that are simply not accessible using the more common late-stage chemistry9,12. In the clinic, automation improves the
reproducibility of radiotracer synthesis, and helps minimize radiation exposure to radiochemists who frequently operate with GBq amounts of radioactivity13. Most automatic radiosynthesizers
are placed inside (mini)hot cells and are operated remotely via a computer interface. Radiotracer automation also improves traceability, documentation management and compliance with
regulatory requirements for quality control14. There are two prominent examples for the automated synthesis of 89Zr-labeled monoclonal antibodies (89Zr-mAbs)3,15. In both reports, the
radiolabeling reaction relies on the use of a pre-functionalized desferrioxamine-antibody conjugate (DFO-mAb). In 2016, Wright et al_._ performed the 89Zr-radiolabeling of
DFO-Bz-NCS-trastuzumab on a microfluidic chip followed by manual purification. The procedure was performed in 45–60 min and gave an isolated product with sufficient activity yield for
multiple patient doses15. More recently, Poot et al_._ reported the fully automated radiolabeling and purification of 89Zr-mAbs in 20193. In this example, single patient doses of purified
89ZrDFO-_N_-Succ-rituximab and 89ZrDFO-_N_-Succ-cetuximab were produced in 77 min starting from 89Zr-oxalate and the respective pre-functionalized DFO-mAb3. The DFO-mAb radiolabeling
precursors are produced by DFO-conjugation to lysine residues on the protein using reagents bearing the activated ester _N_-hydroxysuccinimide (NHS) or a benzylisothiocyanate (Bz-NCS) group
which form amide and thiourea bonds, respectively16,17. DFO-mAb conjugates are typically prepared in advance, characterized, stored, and radiolabeled on demand, and so far, this holds true
for all automated and manual production of 89Zr-mAbs. In general, this chemistry is extremely successful and has facilitated clinical translation of many 89Zr-mAbs18,19,20,21,22. However,
the necessity to produce, characterize and store the DFO-mAb intermediate presents several technological and financial limitations—synthesizing sufficient material for toxicological studies
is both difficult and expensive—that mean accessing 89Zr-mAbs is not always feasible in smaller nuclear medicine facilities. In addition, the long-term storage of an intermediate can lead to
questions over the stability and shelf-life of the material. Recently, we developed a one-pot route that combines the bioconjugation and radiolabeling steps using new DFO-chelates bearing a
photoactivatable aryl azide group (ArN3)23,24,25,26. Three key features of this alternative photoradiochemical method for making 89Zr-mAbs are: _i_) rapid reaction times that depend on the
rate-limiting photoactivation step; _ii_) high chemical tolerance of the light-induced bioconjugation process with many components of mAb formulation buffers, and _iii_) avoidance of the
need to use pre-functionalized DFO-mAbs. The compatibility of the photolabeling process with common mAb formulation buffers, including high concentrations amino acids (e.g. histidine),
surfactants (polysorbate-80), sugars (α,α-trehalose), antioxidants (ascorbate), and various salts such as phosphate buffered saline (PBS) mean that the chemistry often works without the need
to pre-purify the protein from clinical-grade stocks. This critical difference between our photoradiosynthesis approach and the classic multiple step routes for making 89Zr-mAbs led us to
postulate that it could be feasible to make an automated radiosynthesizer that combines all critical steps required in the manufacture of 89Zr-mAbs, namely, oxalic acid neutralization,
bioconjugation, radiolabeling, purification and sterile formulation (Fig. 1). Here, we report the design, manufacture, and proof-of-concept pre-clinical evaluation of ALISI – a prototype
radiosynthesizer for the fully automated light-induced synthesis of 89Zr-mAbs for immuno-positron emission tomography (PET). An overview of the design and plumbing diagram of the ALISI
system is presented in Fig. 2 with full descriptions given in the Methods section. RESULTS Prior to developing the fully automated synthesis of 89Zr-mAbs, we first tested different aspects
of the ALISI system by using model reactions involving the bioconjugation between human serum albumin (HSA) and three photoactivatable compounds. The compounds used were RhodB-PEG3-ArN3,
pre-radiolabeled 68GaDFO-PEG3-ArN3, and the free ligand (DFO-PEG3-ArN3) together with 89Zr-oxalate which forms 89ZrDFO-PEG3-ArN3 in situ. Chemical structures are shown in Figs. 1 and 3a, and
reaction details are presented in Supplemental Tables 1–3. Photochemical activation kinetics were measured to assess the efficiency of the newly designed photoreactor by irradiation of a
stock solution of DFO-PEG3-ArN3. Samples were analyzed by reverse-phase high-performance liquid chromatography (HPLC). The irreversible photoactivation of DFO-PEG3-ArN3 was complete within
90 s, as indicated by full consumption of the starting material in HPLC (Fig. 3b). The three model reactions were used to assess different aspects of the automated system. First, the
photoactivatable fluorophore RhodB-PEG3-ArN3 was used to optimize the sequence and timing of the different steps in the automation protocol. The use of a highly colored (pink)
RhodB-PEG3-ArN3 dye also helped to evaluate the completeness of all reagent transfers by visual tracking and by quantitative measurements of dilution factors using electronic absorption
spectroscopy. We discovered that the lipophilic nature of the small-molecule RhodB-PEG3-ArN3 compound, and the related photolyzed products, means that these species stick to Sephadex columns
which are typically used for protein purification. Consequently, the fluorescently labeled RhodB-PEG3-azepin-HSA product can be purified easily from the small-molecule byproducts on the
ALISI system by using standard PD-10 columns but this model fluorophore was not ideal for optimizing the purification procedure for radiolabeled proteins. Next, test reactions between HSA
and pre-radiolabeled 68GaDFO-PEG3-ArN3 were used to optimize the photochemical conjugation step. Optimization was performed by maximizing the decay-corrected radiochemical conversion (RCC,
measure by size-exclusion chromatography high-performance liquid chromatography, SEC-HPLC) to the labeled protein, 68GaDFO-PEG3-azepin-HSA. We found that pH is a critical factor in
determining the yield of photo-induced bioconjugation reactions using ArN3 species27. Therefore, we investigated the use of different buffers with the aim of controlling pH throughout all
synthetic steps. 2-[4-(2-hydroxyethyl)piperazin-1-yl]ethanesulfonic acid (HEPES) is a zwitterionic buffer commonly used in the synthesis or radiolabeled mAbs17. In our hands, the use of
HEPES at concentrations ≥ 0.5 M had a detrimental impact on the RCC of 68GaDFO-PEG3-azepin-HSA (data not shown). In contrast, reactions in sodium borate buffer (0.25 M, pH8) did not quench
the light-induced conjugation reaction. Experimental data showing the measured RCC _versus_ reaction pH are presented in Fig. 3c. These data show a trend toward increased RCC in the pH
window from 8.0-to-9.5, with RCC reaching 47% between pH8.0–8.7. Below pH8.0, conjugation yields decrease sharply due to progressive protonation of lysine residues. Based on these data,
further reaction parameters were optimized at pH8.0. Our goal was to minimize the manual handling of radioactive components by producing 89Zr-mAbs directly from 89Zr-oxalate stocks and the
unfunctionalized protein. Therefore, we examined the complexation reaction to form 89ZrDFO-PEG3-ArN3 on ALISI (Fig. 3d)24,25. 89Zr-oxalate was transferred into the reaction vial, and then
neutralized by automatic transfer of the Na2CO3 and sodium borate solutions. Then, the DFO-PEG3-ArN3 solution was transferred into the magnetically stirred vial, and 10 s after addition, an
aliquot of the reaction mixture was removed manually with a pipette and spotted immediately onto a silica gel instant-thin-layer chromatography (ITLC) strip (developed with 50 mM DTPA,
pH7.4). The radio-ITLC data showed quantitative radiochemical conversion to give 89ZrDFO-PEG3-ArN3 (which is retained at the baseline, _R_f = 0.0) in < 10 s. In contrast to radiolabeling
of pre-functionalized DFO-mAbs which requires > 45 min, the high diffusion coefficient of the small-molecule components and gentle stirring of the reaction mixture facilitate rapid
89Zr-complexation by DFO-PEG3-ArN3. Data in Fig. 3e show the effect of changing the protein concentration on the measured photochemical conversion yields (PCY) for the synthesis of
RhodB-PEG3-azepin-HSA (purple), or RCC yields for 68GaDFO-PEG3-azepin-HSA (blue) and 89ZrDFO-PEG3-azepin-HSA (black). As with many bioconjugation reactions, photo-induced labeling using ArN3
compounds show a steep dependence on the initial concentration of protein. Conjugation yields began to decrease when [HSA] < 10 mg mL-1. For each of the RhodB-PEG3-azepin-HSA (purple
trace), 68GaDFO-PEG3-azepin-HSA (blue), and 89ZrDFO-PEG3-azepin-HSA products, maximum conjugation yields were obtained at [HSA] ~ 15 mg mL-1 with values of 34.2 ± 0.9%, 49.1 ± 3.9% and 62.9
± 2.2%, respectively. Analysis by size-exclusion chromatography coupled to a high-performance liquid chromatography system (SEC-HPLC, Fig. 3f) confirmed the successful protein-ligation by
co-elution of the HSA protein (orange trace) with that of RhodB-PEG3-azepin-HSA (purple trace, monitoring the fluorophore at 568 nm), and the radioactive traces of 68GaDFO-PEG3-azepin-HSA
(blue trace), and 89ZrDFO-PEG3-azepin-HSA (black trace). The purification of 89Zr-mAbs typically involves using PD-10 desalting columns (Sephadex G-25, 5 kDa exclusion limit) which only work
well when very high RCCs are obtained, or when the small-molecule byproducts are trapped on the Sephadex (as with the RhodB-PEG3-azepin-HSA synthesis, vide supra). However, when RCCs drop
below ~ 80%, the separation efficiency of the PD-10 matrix is sub-optimal. To enhance the radiochemical purity (RCP) of the purified 89Zr-labeled proteins, we constructed custom-made SK-10
columns that have the same geometry as PD-10 columns but are loaded with Sephadex® G-100 (150 kDa exclusion limit) that increases the resolving power. SK-10 columns had a dead volume of ~ 2
mL followed by a protein collection volume of 2.5 mL (Fig. 4a). Small-molecules were effectively retained on SK-10 columns, where a peak-to-peak separation between the desired
89ZrDFO-PEG3-azepin-trastuzumab and the byproducts was > 3 mL, giving a final RCP of the purified protein fraction of > 99% starting from a crude reaction mixture that had an RCC of
42%. Next, we investigated the fully automated production of 89ZrDFO-PEG3-azepin-trastuzumab using ALISI (Supplemental Table 4). Again, changing the protein concentration had an impact on
both decay-corrected RCC for the protein conjugation step and on the isolated decay-corrected RCY of 89ZrDFO-PEG3-azepin-trastuzumab where a plateau was observed in the protein concentration
range of 10–15 mg mL-1 (Fig. 4b). The same trend observed in reactions with HSA was seen with Herceptin. Automation successfully gave purified 89ZrDFO-PEG3-azepin-trastuzumab in < 25 min
starting directly from non-purified Herceptin (14.9 mg mL-1 of protein) with an isolated decay-corrected RCY of 20.1 ± 2.4% (_n_ = 3), and an RCP > 99% (Fig. 4c). Notably, SEC analysis
of the isolated 89ZrDFO-PEG3-azepin-trastuzumab product revealed less than 5% of the activity was associated with an aggregated protein fraction (which elutes at a slightly shorter retention
time). The activity recovered in the purified product was lower than the RCC by a factor of ~ 2. By tracking the activity along the fluid pathway, we found that 89Zr-oxalate was loaded into
the reaction vial with 82.7 ± 3.1% efficiency. The photochemical conjugation efficiency is given by the RCC (~ 45%) and loading of the crude reaction mixture onto the SK-10 column had a
transfer efficiency > 99%. The purification step was, on average, 75.0 ± 4.3% efficient, while sterile filtration led to a small loss in protein-bound activity with a recovered activity
efficiency of 87.7 ± 2.8%. Transfer losses account for the difference between the measured RCC and RCY values. Qualitative testing of the 89ZrDFO-PEG3-azepin-trastuzumab products for
residual borate was performed by adding a few drops of an ethanolic solution of curcumin, which produces a red/orange solution due to the formation of rosocyanine dye in the presence of
borate species28. Visual detection is possible at borate concentrations down to ~ 2.5 mM and tests confirmed that none of the isolated samples of 89ZrDFO-PEG3-azepin-trastuzumab produced by
ALISI contained residual sodium borate. In the clinic, patients undergoing PET scans with 89Zr-mAbs typically receive a dose of 37 MBq of activity administered with between 3 – 100 mg of
total protein29,30,31. In scaled-up syntheses (Supplemental Table S4), we demonstrated the potential of ALISI to produce individual patient doses of 89ZrDFO-PEG3-azepin-trastuzumab. Starting
from 152 MBq of 89Zr-oxalate and 9.7 mg of trastuzumab (formulated as Herceptin), photoradiosynthesis gave an activity yield of 27.9 MBq of isolated 89ZrDFO-PEG3-azepin-trastuzumab with a
decay corrected RCY of 18.3%, an RCP > 99%, a chemical purity > 99%, and a molar activity _A_m of 0.43 MBq nmol-1 of protein. If the ALISI system was qualified for use in a clinical
radiopharmacy, this reaction product would be sufficient to image a patient. Finally, to illustrate the flexibility of the ALISI radiosynthesizer, we adapted the system to automate the
radiolabeling and purification of 89Zr-mAbs via the conventional two-step approach (Fig. 5a). First, trastuzumab was recovered as a purified protein from a clinical-grade sample of
formulated Herceptin by using PD-10 gel filtration. Next, the functionalized DFO-Bz-NCS-trastuzumab radiolabeling precursor was produced in accordance with the methods of Vosjan et al.16,17.
Finally, 89Zr-radiolabeling and automated PD-10 purification using ALISI gave 89ZrDFO-Bz-NCS-trastuzumab with a decay-corrected isolated RCY of 48.3 ± 8.4% (_n_ = 3), an RCP > 99%, and
chemical purity > 99% (Supplemental Table S5) in 90 min. Corresponding radio-ITLC and SEC-HPLC characterization data for 89ZrDFO-Bz-NCS-trastuzumab are shown in Fig. 5b and c,
respectively. Importantly, the purified sample of 89ZrDFO-Bz-NCS-trastuzumab contained ~ 15% of 89Zr-activity associated with an aggregated protein peak. This is common feature of the
conventional two-step radiolabeling method used in the clinic, which requires removal of the mAb from the stabilizing formulation components prior to functionalization with DFO-Bz-NCS23. The
presence of 89Zr-labeled protein aggregate is associated with accumulation of activity in the liver and spleen. In comparison, the photoradiochemical approach reduces this aggregate
fraction by a factor of ~ 3 which would likely improve image contrast and reduce the radiation burden to the patient. DISCUSSION The ALISI radiosynthesizer was constructed by using a
combination of open-source microcontrollers (Arduino) and computer-aided design (CAD), coupled with additive manufacturing32,33. Arduino electronic components are modular and expandable.
This allows for rapid and facile integration of new features during prototype development. Liquid handling components and all fluidic pathways employ commercially available, single-use,
sterile tubing and three-way switching valves operated by digital servomotors. These components were chosen to facilitate future translation of ALISI to a clinical environment. The
custom-made, electropolished photoreactor houses three high-powered LEDs with peak emission at 365 nm (Fig. 2c). Light-induced activation of our ArN3 reagents is rate-limiting in the
photoradiosynthesis of 89Zr-mAbs, and in comparison with manual reactions, the mirrored photoreactor of ALISI gave a 5-to-10-fold decrease in reaction time26. It is important to note that
the bioconjugation efficiency (as measured from the PCY or RCC values) shows a strong dependence on the reaction geometry and the conditions used. For instance, the nature of the photoactive
compound and protein substrate, the choice of radionuclide, the concentrations of all reagents, the buffer composition, and the pH influence conjugation efficiency. Under identical
conditions, RhodB-PEG3-ArN3 gave consistently lower yields for HSA labeling than pre-radiolabeled 68GaDFO-PEG3-ArN3, which in-turn, was lower than radiolabeling with 89ZrDFO-PEG3-ArN3
generated in situ. Under our optimized conditions, the observed bioconjugation yields using an initial 1:1 stoichiometric ratio between HSA and the photoactive compound were in the range of
35%–65%. Standard bioconjugation reactions used to make the DFO-mAbs, including activated ester16 or thiourea17 chemistry, have efficiencies in the range of ~ 20%–75% (see Supplementary
Methods). These data confirm that photo-induced bioconjugation is equally successful compared to current state-of-the-art methods used to make radiolabeled mAbs in clinical practice. For the
photoradiosynthesis of 89ZrDFO-PEG3-azepin-trastuzumab from Herceptin, the RCC was ~ 45%. This is remarkable considering that the radiolabeling and bioconjugation steps are complete in <
90 s, and the reaction uses non-purified mAb where the mixture contains all formulation components of clinical-grade Herceptin. We note that radiochemists performing manual syntheses of
89Zr-mAbs are familiar with obtaining near quantitative RCYs, but in most cases, the reaction only involves the radiolabeling step (not the conjugation). Since test reactions are usually
employed to predetermine the maximum molar activity of a sample, quantitative labeling is expected. In a recent study, Poot et al. automated the radiolabeling and purification of 89Zr-mAbs
from pre-functionalized DFO-mAbs with RCYs in the range 60%–75%3. With the present photochemistry, formation of 89ZrDFO-PEG3-ArN3 in situ is quantitative and the decay-corrected isolated RCY
of 89ZrDFO-PEG3-azepin-trasutzumab (20.1 ± 2.4%) encompasses all chemical reactions, transfers, and processing steps. Transfer losses are one of the main limitations of adapting manual
chemistry to automated platforms. Considering the multi-step nature of the ALISI protocol, which includes all reagent transfers, acid neutralization, buffer control, radiolabeling and
bioconjugation, in-line purification, sterile filtration, and product formulation, the observed RCYs are an excellent benchmark. Purification using SK-10 columns filled with Sephadex® G-100
media provided greatly enhanced separation and improved RCP (> 99%) of the isolated 89ZrDFO-PEG3-azepin-trasutzumab when compared with PD-10 columns. This is expected since the Sephadex
G-25 media used in PD-10 columns is primarily intended for desalting and is sub-optimal for separating large proteins from small-molecule components. Finally, automated radiosynthesis of
89Zr-mAbs on ALISI improved the reproducibility of the chemistry by standardizing the reaction geometry and conditions, and by decreasing the potential for user-related, irreproducible
errors. Collectively, the successful synthesis and isolation of 89ZrDFO-PEG3-azepin-trastuzumab using our automated radiosynthesizer suggest that ALISI is potentially useful for preparing
individual patient doses or small batches of 89Zr-mAbs (and other radiolabeled proteins) on demand. With further development, we anticipate that the system can be adapted for use with other
radionuclides and photoactivatable chelates to access radiopharmaceuticals for applications in radioimmunotherapy. CONCLUSION We developed a radiosynthesizer unit that performs the fully
automated light-induced synthesis of 89Zr-mAbs for immunoPET. The ALISI system can produce 89Zr-mAbs in < 25 min in high radiochemical purity (RCP > 99%) starting from stock solutions
of 89Zr-oxalate, a photoactivatable DFO-PEG3-ArN3 chelate, and a protein of interest. Features of the automated protocol include neutralization of the oxalic acid, buffer exchange and pH
correction, quantitative formation of the photoactivatable 89ZrDFO-PEG3-ArN3 complex, rapid (< 90 s) light-induced protein-ligation, in-line purification using novel SK-10 size-exclusion
chromatography columns, and finally sterile filtration and product formulation in a biocompatible medium. After loading the reagent reservoirs, all steps are performed at the touch of a
single button. The system is also highly flexible, producing purified 89Zr-mAbs via conventional labeling of a pre-functionalized DFO-mAb conjugate. With further development, we anticipate
that ALISI could facilitate on-demand access to individual patient doses of 89Zr-mAbs in Nuclear Medicine facilities that rely on automated devices for radiotracer production. METHODS
Further details are presented in the Supplemental Materials. Schematics and sample code for operating the microcontrollers are available on request. PROTEIN SAMPLES Formulated Herceptin™
(Roche/Genentech, South San Francisco, CA) and human serum albumin (HSA; Merck, Darmstadt, Germany) were reconstituted in water (> 18.2MΩ·cm at 25 °C). Protein concentrations were
determined by using a Nanodrop™ OneC Microvolume UV–Vis Spectrophotometer. ADDITIVE MANUFACTURING The ALISI radiosynthesizer was constructed by using the computer assisted design (CAD)
software Solidworks2020 (Dassault Systèmes, Vélizy-Villacoublay, France). Components were prepared through additive manufacturing, laser cutting or purchased directly from commercial
vendors. Additive manufacturing components were produced by selective laser sintering and made from PA2200, a fine powder based on polyamide-12. The synthesizer case was manufactured from
laser cut, 5 mm plates of high tensile strength, black polyoxymethylene (POM) and an aluminum profile modular assembly system (Kanya AG, Rüti, Switzerland). CONTROL AND ELECTRONICS
Electronic components are based around the single-chip ATmega328 microcontroller. Microcontrollers, breakout boards, additional circuit boards, and electronic components were either
custom-manufactured or purchased from Arduino.cc (Ivrea, Italy), Adafruit Industries (New York City, NY, USA), RS Components (Frankfurt-am-Main, Deutschland), or Distrelec AG (Nänikon,
Switzerland). The photoreactor consists of an electropolished stainless steel tube containing an array of three, high-powered light-emitting diodes (LEDs; Nichia, Anan, Japan) with a light
output of 1.03 W per LED at 365 nm. Valve modulation is performed with standard digital servos (Savöx, Salt Lake City, UT, USA). All liquid transfer steps are driven pneumatically with a
syringe pump controlled by a NEMA-17 bipolar stepper motor (Distrelec AG, Nänikon, Switzerland). LIQUID HANDLING AND CASSETTES All components for the disposable cassette system were either
custom-manufactured or purchased from commercial vendors (B. Braun Melsungen AG, Meslungen, Germany, or BD, Heidelberg, Germany). The cassettes use flexible, high chemical resistance Tygon
tubing. For purification, custom-made SK-10 separation columns (Econo-Pac chromatography columns, Bio-Rad Laboratories, Hercules, CA, USA) filled with 8.3 mL of pre-soaked Sephadex® G-100
(Merck, Darmstadt, Germany) were constructed. The stationary phase of the SK-10 columns was capped with filter-frits and columns were eluted with sterile PBS (pH7.4). SYNTHESIS AND
RADIOCHEMISTRY The photoactivatable chelate DFO-PEG3-ArN3 and the fluorescent photoactivatable fluorophore (_PhotoTag_) RhodB-PEG3-ArN3 were synthesized and characterized as described
previously24. The stock solution of 89Zr-oxalate ([89Zr(C2O4)4]4-(aq.) in ~ 1 M oxalic acid) was obtained from PerkinElmer (Waltham, MA, USA; manufactured by the BV Cyclotron VU, Amsterdam,
The Netherlands) and was used without further purification. Full experimental details on the model chemistry using RhodB-PEG3-ArN3, 68GaDFO-PEG3-ArN3 and 89Zr-oxalate in combination with
DFO-PEG3-ArN3 are given in the Supplemental Information. All reported RCC and RCY values are decay corrected. SETUP FOR AUTOMATED RADIOLABELING Reactions with ALISI were performed on new
liquid handling equipment, freshly assembled from sterile packaging. Briefly, individual reservoirs were assigned to stock solutions of Na2CO3(aq.), DFO-PEG3-ArN3, 89Zr-oxalate, protein,
reaction buffer, and sterile PBS for purification by size-exclusion chromatography (SEC) (see Supplemental Tables 1–4). A minimum volume of ~ 50μL was necessary to achieve adequate liquid
transfer. For test reactions where reagent volumes were below this threshold, the total volume was adjusted with an appropriate volume of water. DESCRIPTION OF THE AUTOMATED
89ZR-PHOTORADIOLABELING PROCEDURE A schematic of the plumbing diagram and a photograph of the ALISI system set-up for the synthesis of 89Zr-mAbs is shown in Fig. 2. The radiosynthesizer unit
is initialized by pushing the power button. After initialization, the system provides a digital prompt on the built-in LCD-display to indicate that the device is ready to start. Automated
radiosynthesis and purification is initiated by pressing the start button. Thereafter, the radiosynthesizer transfers all reagents and components to the reaction vial, located inside the
photoreactor, in the following sequence: _i_) 89Zr-oxalate in ~ 1 M oxalic acid is transferred. _ii_) An equal volume of 1 M Na2CO3(aq.) is transferred. _iii_) DFO-PEG3-ArN3 in a solution of
~ 10% DMSO and aqueous sodium borate buffer (0.25 M, pH8.0) is removed from the reservoir and first used to wash the 89Zr-stock solution reservoir before being delivered to the reaction
vial. _iv_) The protein solution is transferred. _v_) Additional sodium borate buffer (0.25 M, pH8.0) is used to wash the protein reservoir and then transferred. After the reagent transfer
sequence, the reaction mixture is irradiated with 365 nm light for 90 s. Tests indicated that the temperature of the reaction mixture does not change during this time, but the high-powered
LEDs require cooling with an aluminum heat-sink attached to a fan. After irradiation, the crude mixture is transferred to the SK-10 size-exclusion column (SEC) for purification. After
automatic separation, the product fraction containing the high molecular weight protein is filtered through a standard 0.22 μm sterile filter and collected in a sterile vial. The product is
formulated in sterile PBS (pH7.4), and after quality control, is ready for use in radiochemical, cellular, or in vivo assays. DATA ANALYSIS Data were plotted by using the GraphPad Prism 9.0
software (GraphPad Software Inc., San Diego, California USA). DATA AND CODE AVAILABILITY STATEMENT An electronic computer-aided design file containing the full assembly and all components of
the radiosynthesizer required for 3D-printing, as well as the Arduino program (sketch) is available from the corresponding author. REFERENCES * Lin, M. _et al._ Fully automated preparation
of 68Ga-PSMA-11 at curie level quantity using cyclotron-produced 68Ga for clinical applications. _Appl. Radiat. Isot._ 155, 108936 (2020). Article CAS Google Scholar * Thisgaard, H. _et
al._ Multi-curie production of gallium-68 on a biomedical cyclotron and automated radiolabelling of PSMA-11 and DOTATATE. _EJNMMI Radiopharm. Chem._ 6, 1 (2021). Article Google Scholar *
Poot, A. J. _et al._ Fully automated 89Zr labeling and purification of antibodies. _J. Nucl. Med._ 60, 691–695 (2019). Article CAS Google Scholar * Wichmann, C. W., Ackermann, U. &
Poniger, S. Automated radiosynthesis of [68Ga]Ga-PSMA-11 and [177Lu]Lu-PSMA-617 on the iPHASE MultiSyn module for clinical applications. _J. Label Compd. Radiopharm._ 64, 140–146 (2020).
Article Google Scholar * Collet, C. _et al._ Fully automated radiosynthesis of [18F]fluoro-C-glyco-c(RGDfC): exploiting all the abilities of the All-In-One synthesizer. _React Chem. Eng._
4, 2088–2098 (2019). Article CAS Google Scholar * Davis, R. A., Drake, C., Ippisch, R. C., Moore, M. & Sutcliffe, J. L. Fully automated peptide radiolabeling from [18F]fluoride. _RSC
Adv._ 9, 8638–8649 (2019). Article CAS Google Scholar * Collier, T. L. _et al._ Synthesis and preliminary PET imaging of 11C and 18F isotopologues of the ROS1/ALK inhibitor lorlatinib.
_Nat. Commun._ 8, 15761 (2017). Article CAS Google Scholar * Miller, P. W., Long, N. J., Vilar, R. & Gee, A. D. Synthesis of 11C, 18F, 15O, and 13N radiolabels for positron emission
tomography. _Angew Chemie Int. Ed._ 47, 8998–9033 (2008). Article CAS Google Scholar * Liang, S. H. & Vasdev, N. Total Radiosynthesis: Thinking Outside ‘the Box’’’. _Aust. J. Chem._
68, 1319–1328 (2015). Article CAS Google Scholar * Rotstein, B. H. _et al._ 11CO2 fixation: A renaissance in PET radiochemistry. _Chem. Commun._ 49, 5621–5629 (2013). Article CAS Google
Scholar * Jacobson, O., Kiesewetter, D. O. & Chen, X. Fluorine-18 radiochemistry, labeling strategies and synthetic routes. _Bioconjug. Chem._ 26, 1–18 (2015). Article CAS Google
Scholar * Hicks, J. W. _et al._ Towards the preparation of radiolabeled 1-aryl-3-benzyl ureas: Radiosynthesis of [11C-carbonyl] AR-A014418 by [11C] CO2 fixation. _Bioorg. Med. Chem. Lett._
22, 2099–2101 (2012). Article CAS Google Scholar * Kaplan, D. J., Patel, J. N., Liporace, F. A. & Yoon, R. S. Intraoperative radiation safety in orthopaedics: A review of the ALARA
(As low as reasonably achievable) principle. _Patient Saf. Surg._ 10, 1–7 (2016). Article Google Scholar * Thompson, S., Kilbourn, M. R. & Scott, P. J. H. Radiochemistry, PET imaging,
and the Internet of chemical things. _ACS Cent. Sci._ 2, 497–505 (2016). Article CAS Google Scholar * Wright, B. D. _et al._ Microfluidic preparation of a 89Zr-Labeled Trastuzumab
single-patient dose. _J. Nucl. Med._ 57, 747–752 (2016). Article CAS Google Scholar * Verel, I. _et al._ 89Zr Immuno-PET: comprehensive procedures for the production of 89Zr-Labeled
monoclonal antibodies. _J. Nucl. Med._ 44, 1271–1281 (2003). CAS PubMed Google Scholar * Vosjan, M. J. W. D. _et al._ Conjugation and radiolabeling of monoclonal antibodies with
zirconium-89 for PET imaging using the bifunctional chelate p -isothiocyanatobenzyl- desferrioxamine. _Nat. Protoc._ 5, 739–743 (2010). Article CAS Google Scholar * Deri, M. A., Zeglis,
B. M., Francesconi, L. C. & Lewis, J. S. PET imaging with 89Zr: From radiochemistry to the clinic. _Nucl. Med. Biol._ 40, 3–14 (2013). Article CAS Google Scholar * Henry, K. E.,
Ulaner, G. A. & Lewis, J. S. Human epidermal growth Factor Receptor 2-Targeted PET/Single- Photon emission computed tomography imaging of breast cancer. _PET Clin._ 12, 269–288 (2017).
Article Google Scholar * Lohrmann, C. _et al._ Retooling a blood-based biomarker: Phase I assessment of the high-affinity CA19-9 antibody HuMAB-5B1 for immuno-PET imaging of pancreatic
cancer. _Clin Cancer Res._ 25, 7014–7023 (2019). Article CAS Google Scholar * Berg, E. _et al._ Total-Body PET and highly stable chelators together enable meaningful 89Zr-Antibody PET
studies up to 30 days after injection. _J. Nucl. Med._ 61, 453–460 (2020). Article CAS Google Scholar * Nemieboka, B. _et al._ Radiopharmacologic screening of antibodies to the unshed
ectodomain of MUC16 in ovarian cancer identifies a lead candidate for clinical translation. _Nucl. Med. Biol._ 86–87, 9–19 (2020). Article Google Scholar * Klingler, S., Fay, R. &
Holland, J. P. Light-induced radiosynthesis of 89Zr-DFO-Azepin-Onartuzumab for imaging the hepatocyte growth factor receptor. _J. Nucl. Med._ 61, 1072–1078 (2020). Article CAS Google
Scholar * Guillou, A., Earley, D. F. & Holland, J. P. Light-activated protein-conjugation and 89Zr-radiolabelling with water-soluble desferrioxamine derivatives. _Chem. Eur. J._ 26,
7185–7189 (2020). Article CAS Google Scholar * Guillou, A., Earley, D. F., Patra, M. & Holland, J. P. Light-induced synthesis of protein conjugates and its application in
photoradiosynthesis of 89Zr-radiolabeled monoclonal antibodies. _Nat Protoc._ 15, 3579–3594 (2020). Article CAS Google Scholar * Patra, M., Klingler, S., Eichenberger, L. S. &
Holland, J. P. Simultaneous photoradiochemical labeling of antibodies for immuno-positron emission tomography. _iScience_ 13, 416–431 (2019). Article CAS Google Scholar * Patra, M.,
Eichenberger, L. S., Fischer, G. & Holland, J. P. Photochemical conjugation and one-pot radiolabelling of antibodies for Immuno-PET. _Angew Chemie Int Ed._ 58, 1928–1933 (2019). Article
CAS Google Scholar * Spicer GS, Strickland JDH. 907. Compounds of curcumin and boric acid. Part II. The structure of rubrocurcumin. _J. Chem. Soc_. 4650–4653 (1952). * Dijkers, E. C. _et
al._ Biodistribution of 89Zr-trastuzumab and PET imaging of HER2-positive lesions in patients with metastatic breast cancer. _Clin. Pharmacol. Ther._ 87, 586–592 (2010). Article CAS
Google Scholar * Bensch, F. _et al._ Comparative biodistribution analysis across four different 89Zr-monoclonal antibody tracers-The first step towards an imaging warehouse. _Theranostics._
8, 4295–4304 (2018). Article CAS Google Scholar * Bensch, F. _et al._ 89Zr-trastuzumab PET supports clinical decision making in breast cancer patients, when HER2 status cannot be
determined by standard work up. _Eur. J. Nucl. Med. Mol. Imaging._ 45, 2300–2306 (2018). Article CAS Google Scholar * Badamasi Y. A. The working principle of an Arduino. In _2014 11th
International Conference on Electronics, Computer and Computation (ICECCO)_. IEEE:1–4 (2014). * Rossi, S., Puglisi, A. & Benaglia, M. Additive manufacturing technologies: 3D printing in
organic synthesis. _ChemCatChem_ 10, 1512–1525 (2018). Article CAS Google Scholar Download references ACKNOWLEDGEMENTS We thank the Swiss National Science Foundation (SNSF Professorship
PP00P2_163683 and PP00P2_190093), the Swiss Cancer Research Foundation (KFS-4257-08-2017), and the University of Zurich (UZH) for financial support. This project has received funding from
the European Research Council under the Grant Agreement numbers 676904, ERC-StG-2015, NanoSCAN and 101001734, ERC-CoG-2020, PhotoPHARMA. We thank Dr Amaury Guillou for synthesis of the
photoactivatable compounds. We thank Sascha Weidner and the AddMan Factory at the University of Zurich. AUTHOR INFORMATION AUTHORS AND AFFILIATIONS * Department of Chemistry, University of
Zurich, Winterthurerstrasse 190, 8057, Zurich, Switzerland Simon Klingler & Jason P. Holland Authors * Simon Klingler View author publications You can also search for this author
inPubMed Google Scholar * Jason P. Holland View author publications You can also search for this author inPubMed Google Scholar CONTRIBUTIONS S.K. and J.P.H. designed the project. S.K.
performed all experiments. S.K. and J.P.H. wrote the manuscript. J.P.H supervised the project. CORRESPONDING AUTHOR Correspondence to Jason P. Holland. ETHICS DECLARATIONS COMPETING
INTERESTS The authors declare no competing interests. ADDITIONAL INFORMATION PUBLISHER'S NOTE Springer Nature remains neutral with regard to jurisdictional claims in published maps and
institutional affiliations. SUPPLEMENTARY INFORMATION SUPPLEMENTARY INFORMATION. RIGHTS AND PERMISSIONS OPEN ACCESS This article is licensed under a Creative Commons Attribution 4.0
International License, which permits use, sharing, adaptation, distribution and reproduction in any medium or format, as long as you give appropriate credit to the original author(s) and the
source, provide a link to the Creative Commons licence, and indicate if changes were made. The images or other third party material in this article are included in the article's
Creative Commons licence, unless indicated otherwise in a credit line to the material. If material is not included in the article's Creative Commons licence and your intended use is not
permitted by statutory regulation or exceeds the permitted use, you will need to obtain permission directly from the copyright holder. To view a copy of this licence, visit
http://creativecommons.org/licenses/by/4.0/. Reprints and permissions ABOUT THIS ARTICLE CITE THIS ARTICLE Klingler, S., Holland, J.P. Automated light-induced synthesis of 89Zr-radiolabeled
antibodies for immuno-positron emission tomography. _Sci Rep_ 12, 668 (2022). https://doi.org/10.1038/s41598-021-04626-5 Download citation * Received: 27 September 2021 * Accepted: 28
December 2021 * Published: 13 January 2022 * DOI: https://doi.org/10.1038/s41598-021-04626-5 SHARE THIS ARTICLE Anyone you share the following link with will be able to read this content:
Get shareable link Sorry, a shareable link is not currently available for this article. Copy to clipboard Provided by the Springer Nature SharedIt content-sharing initiative