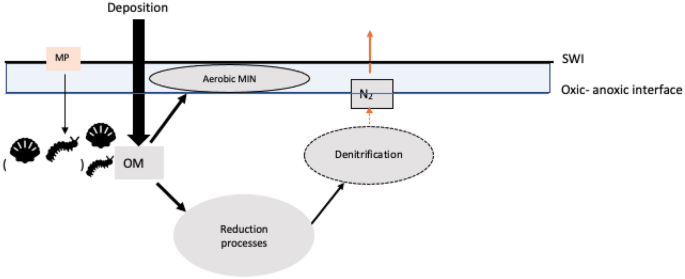
Modelled broad-scale shifts on seafloor ecosystem functioning due to microplastic impacts on bioturbation
- Select a language for the TTS:
- UK English Female
- UK English Male
- US English Female
- US English Male
- Australian Female
- Australian Male
- Language selected: (auto detect) - EN
Play all audios:

ABSTRACT Bioturbating species play an essential role in regulating nutrient cycling in marine sediments, but their interaction with microplastics (MP) remains poorly understood. Here we
investigated the linkage between MP and ecosystem functioning using experimental observations of luminophore distribution in the sediment to parametrize bioturbation coefficients (Db). this
information as fed into a simplified transport-reaction model, allowing us to upscale our experimental results. We found that the composition of bioturbators modulated shifts in the
ecosystem functioning under microplastic stress. Maldanid worms (_Macroclymenella stewartensis)_, functionally deep burrowing and upward-conveyor belt feeders, became less active. The Db of
_M. stewartensis_ reduced by 25% with the addition of 0.002 g MP cm−2 at surface sediment, causing accumulation of organic matter in the oxic sediment zone and stimulating aerobic
respiration by 18%. In contract, the tellinid bivalve _Macomona liliana,_ functionally a surface -deposit feeder that excretes at depth_,_ maintained particle mixing behaviour in
MP-contaminated systems. This study provides a mechanistic insight into the impacts of MP and indicates that the functional role of bioturbating species should be involved in assessing the
global impact of MP. The model allowed us to understand the broad-scale impact of MP on seafloor habitat. SIMILAR CONTENT BEING VIEWED BY OTHERS IMPACTS OF WARMING AND NUTRIENT ENRICHMENT ON
THE FATE AND EFFECTS OF NANOPLASTICS IN A FRESHWATER FOOD WEB Article 01 November 2024 BENTHIC REMINERALIZATION UNDER FUTURE ARCTIC CONDITIONS AND EVALUATING THE POTENTIAL FOR CHANGES IN
CARBON SEQUESTRATION IN WARMING SEDIMENTS Article Open access 07 October 2024 MICROENVIRONMENTS ON INDIVIDUAL SAND GRAINS ENHANCE NITROGEN LOSS IN COASTAL SEDIMENTS Article Open access 11
May 2025 INTRODUCTION Plastics of micro- and nano-size are the most hazardous and wide-spread plastic pollution1,2,3, but their ecological impacts involve complex interactions among
environmental components that are largely unknown 4, 5. Most microplastics (MP, dia. < 0.5 cm) end up on the seafloor, particularly in coastal sediments6,7,8,9. Plastic particles
deposited in sediment habitats are threatening benthic species. For example, the polychaete _Arenicola marina_ suffered a 50% loss of energy reserves with reduced feeding rates when they
ingested unplasticized polyvinylchloride (UPVC) particles10. Green et al. (2016) demonstrated that after one-month exposure _A. marina’s_ burrowing capacity decreased with increasing dosages
of MP (from 0.02%, 0.2%, 2% by wet weight sediment)11. The deposit-feeding bivalve _Macomona liliana_ lost burrow capacity after 31-day exposure to polyethylene terephthalate microplastics
(PET) (1% by sediment weight)12. Recent studies found sediment dwellers (e.g., the deposit feeding bivalve _Limecola balthica_) avoids the MP by penetrating deeper to the sediment and
reduces their food intakes13. These effects on worm and bivalve species lead to changes in their behaviours and potential shifts in ecosystem function roles, notably in bioturbation.
Bioturbation is referred as the movement of sediment particles and porewater by animals: it plays a critical functional role of benthic macrofauna mediating the ecosystem responses and
enhances the cycling of carbon and nitrogen in marine environments14,15,16,17. Bioturbation can take many forms depending on animal body-size, density, feeding and burrowing strategies.
These factors affect how animals redistribute particles from the sediment surface to different depths18, 19, influence the sediment erodibility20, 21, determine the heterogeneity of biogenic
habitats, and maintain the resilience of ecosystem functions22, 23. Bioturbation modes also influence how MP transport in the sediments. The conveyor-belt feeders that can transport
particles in both up and downward directions might induce a deep penetration of MP in the sediment, while biodiffusors with strict upward conveying mode retain less MP in the deep zone24.
However, the cumulative effects of MP pollution on bioturbation will depend on the sensitivity of individual species and the specific role they play in the sediment. The loss of large
macrofauna and their functional roles can lead to a cascading effect on the ecosystem functioning25,26,27; therefore, the potential for broad-scale shifts in ecosystem functions due to the
impact of MP on the macrofauna playing a role as bioturbator needs to be assessed28, 29. Here, we upscale the impacts of MP to an ecosystem level by reparametrizing the bioturbation
coefficient Db. Db is derived from the biological mixing patterns that reflect the response of bioturbators to their environmental conditions (e.g., contamination, sedimentation, food
availability)16, 30. For example, in petrol-contaminated sediments, the benthic infauna community contributed to a peak accumulation of particles 2–4 cm sediment depth31. Similarly, the
polychaete (_Perinereis adbuhitensis_) lost its deep transporting ability with particle penetration to a depth of 6–8 cm reduced with the increasing concentrations of cadmium and copper32.
Such observed changes in particle transport profiles are the indicator of pollution stress on infaunal groups. In transport-reaction models 17, 33, 34, the bioturbation coefficient (Db)
represents the macrofaunal function role in reworking sediment particles and transferring organic matter (OM) to the microbial community in the sediment as a critical step for OM
degradation. The penetrated OM stimulates microbial activities at different sediment zones e.g., aerobic mineralization and denitrification 35,36,37. In a simplified sediment system, aerobic
mineralization takes place in the oxic zone34. The anoxic zone is the central place for multiple reduction processes such as NO3- being reduced to NH4+ or denitrifying to N2 38; the reduced
products from anaerobic processes in turn also enhance denitrification17. Potentially, the bioturbation coefficient Db could mediate the impact of MP to ecosystem functions and allow
observations of how MP changes Db to be upscaled to assess broadscale consequences of MP pollution in marine seafloors. In this study, we measured Db in a laboratory experiment investigating
the impacts of MP on two large and functionally important species, the maldanid polychaete _Macroclymenella stewartensis_ and tellinid bivalve _Macomona liliana_. Db measurements made under
different MP concentrations were then used to parameterize a simplified transport-reaction model34 (Table S2, supplementary). We choose this model because it provides estimates of organic
matter (OM) fluxes down the sediment column. Db is a key driver of the redistribution of OM influxes and thus the portion of aerobic mineralization in the oxic sediment34. This model assumes
that, in the oxic zone, the penetrated OM induces equivalent oxygen demands for the complete aerobic degradation. In the anoxic zone, the penetrated OM participates in denitrification
processes, and multiple anaerobic processes deliver the reduced components (e.g., NH4+, Fe2+, Mn2+, H2S) to enhance the N-cycling17, 39. The difference between OM inputs and aerobic
mineralization in this virtual ecosystem provides a proxy for reduction processes. Denitrification consumes energy from penetrated OM to regenerate N2 17. In the context of this study, N2
production is a proxy for reduction processes that omits the complexity of multiple degradation pathways, and it is the source of the N2 released from sediment–water interface (SWI) (Fig.
1). RESULTS BIOTURBATION COEFFICIENT DB VARY IN RESPONSE TO CHANGES IN MP CONCENTRATION In treatments hosting only worms or the combined two species, decreases of Db compared to the control
were 25% with 0.002 g cm−2 of MP (Fig. 2; Table 1). The highest Db value (10.69cm2 year−1) was measured in the worm groups without MP and changes of Db compared to the control was almost
-30% with high concentration level of MP (0.02 g cm−2) (Table 1). Bivalve groups had a relatively stable particle mixing intensity (7.04–7.22 cm2 year−1) in MP treatments. The difference
between worm and bivalve groups on Db values decreased (7.58 cm2 year–1 vs. 7.17 cm2 year−1) when exposed to a higher concentration of MP (0.02 g cm−2). Concentrations of OM penetrated along
sediment depths were determined by Db from bivalve, combined and worm groups separately (Fig. 3). In worm groups, MP treatments had more OM depositing in the sediment surface (0–2 cm). The
group with combined of bivalve and worm had a similar trend. A convergence of OM concentration profiles from bivalve group shows there was no change in OM penetration profiles after adding
MP. ECOSYSTEM FUNCTIONING IN MP-BIOTURBATOR GROUPS Fraction of aerobic mineralization (β) accounting to total OM degradation varied with Db in the worm-only and combined groups, and this
induced changes on oxygen consumption and nitrogen production (Fig. 4; Table 2). In the worm groups, aerobic mineralization fraction increased when Db decreased: the percentage of change of
O2 consumption rates was from 6.58 to 18.73% when MP concentration increased from 0.0002 to 0.02 g cm−2 (Fig. 4; Table 2). N2 production compared to the control decreased from 1.58 to 4.49%
at the expense of increased aerobic mineralization. A similar, but weaker trend was in the combined groups: when MP concentration reached 0.002 g cm−2, the O2 consumption rates compared to
the control increased by 15.66%. The fraction of aerobic mineralization β, oxygen consumption rates F_o2 and estimated N2 production R(N) were invariant in bivalve groups when MP
concentration increased. DISCUSSION BIOTURBATION COEFFICIENTS IN MP-CONTAMINATED SYSTEM We expect that the functional traits of different species, such as the feeding and movement of worms
and bivalves determine the responses of the sediment ecosystem to MP pollution. In our study, the highest Db was observed in experiments occupied by worms without MP and this value decreased
as MP concentrations increased, whereas the Db of bivalves was relatively stable. As a tube builder, the maldanid polychaete _M. stewartensis_ feeds head-down around 10 cm depth in the
sediment and excretes faeces on the sediment surface40. This burrowing mode enlarges turnover areas through sediment columns, increasing the oxygen penetration depth and subducting more
labile OM to the deep zone 41, 42. This behaviour can explain higher bioturbation coefficients in the worm groups compared to the bivalve ones. Exposure to MP increases the risk of worms
ingesting MP, and this may cause health issues including gut inflammation, reduction of their ingestion rates and energy reserves, suppression of burrowing activities, and limitation to
reproduction10, 11. The Db values of worms decreased to a level similar to the bivalve’s when MP concentration was increased. A reduced bioturbation rate means less sediment turnover scales
and particle mixing43. While our results imply potential toxic effects of MP on maldanid burrowing behaviour, this is not apparent for the deposit-feeding bivalve (_M. liliana_) which uses a
long siphon to capture the food particles at the sediment surface44. Previous studies have shown that _M. liliana_ are impacted by MP with reduced reburial rates12, 45 which seem to
contrast our finding in terms of bioturbation. However, in this study we focused on particle movement to calculate Db, whereas the role of _M. liliana_ in regulating the nutrient cycling may
be more strongly linked to porewater advection and redox oscillations46. _M. liliana_ periodically pressures overlying fluids and oxygenized-water to the burrowed zone during feeding
period47. Although the particle reworking intensity of the bivalve group in MP treatments remains stable, it might be because the parameter Db has limitations in explaining these fluid
oscillation behaviours. MP weakened the particle mixing intensity by maldanid worm and reworking behaviours of tellinid bivalve might dominate the system when MP concentration increased. Db
values from worm groups decreased by the increased concentration of MP; the values from bivalve and combined groups converged to a similar level (7.22, 7.04 cm2 year−1) when MP concentration
reached 0.002 g cm−2. Chemical pollution has been reported to reduce the bioturbation potential as a result of the losing species diversity and reduced community biomass48. Large and
functional important species have dominant impacts on particle redistribution and nutrient cycling as well as the community structure, which is more influential than species diversity25, 27,
49. Bioturbating species take different strategies to adapt to environment stresses (e.g., marine heatwaves, acidification, nutrient loading), with the cascading-effect on ecosystem
functioning depends on the functional traits of key species50,51,52. Therefore, feedback from the ecosystem (e.g., nutrient fluxes and oxygen consumption) associated with sediment reworking
will depend on the responses from relative dominant species to MP and the specific mechanisms by which they fulfil their individual and collective functional roles. In this study, changes of
Db reflect a potentially different impact of MP on the sediment reworking in the areas dominant with maldanid worm _M. stewartensis_ and tellinid bivalve _M. liliana_ separately, as well as
their transition zones (co-occur of these species) in the seafloor habitats. The bioturbation of _M. liliana_ and _M. stewartensis_ creates distinct microtopographic features on the
sediment surface that influence the nutrient fluxes53. In MP-contaminated habitats, the maldanid worm can loss advantage in deep-particle mixing and maintaining the nutrient cycling.
Functional traits of _M. liliana_ associated with sediment reworking and generation of porewater pressure gradients will lead the ecosystem processes when two species co-occur, and the
habitats becomes homogenized regarding the loss of worm’s functional roles. This is likely to happen in the natural habitat when the concentration of MP continues to increase. Currently,
there is no consistent way to measure MP concentration, and differences from areal concentrations to mass-based concentrations are common. MP concentrations in the field are often variable,
sites with concentration spikes are associated with the in-situ breakdown of larger plastic items9, 54, 55. The range of MP used in our treatments reflects both the variation between sites
but also the potential extreme values within sites associated with spatial and temporal dynamics56,57,58. Even MP increasing to 0.002 g cm−2 is concerning because the similar contamination
level was found in field studies59. ECOLOGICAL CONSEQUENCES FROM MP-CONTAMINATED SYSTEM Our measurement of Db under different MP loads combined with the application of the transport-reaction
model indicates that in plastic polluted sediments, less organic matter (OM) is subducted deep into the sediment resulting in higher oxygen consumption in the sediment. The associated
effects on ecosystem functioning with bioturbations are summarized in Fig. 5. The shallow penetration of OM stimulates microbial activities in the oxic zone with more oxygen demands for the
respiration. Increasing oxygen demands in MP-contaminated sediments were reported in previous laboratory tests13, and was associated with the reduced burrowing capacity of worms and bivalves
with different functional traits to our study species (i.e., _Arenicola marina_, _Cerastoderma glaucum_)11, 13. The observation from a real-world experiment reveals that mollusc abundance
alone cannot explain the increasing oxygen demands in the dark (aerobic respiration), and the linkage of macrofauna and sediment oxygen consumption can be broken with the contamination of
fibric microplastic60. Our study provides a mechanistic insight into this phenomenon: aerobic-microbial activities consuming OM at the sediment surface may overweigh macrofauna’s mediating
effects on microbial activities through oxic-anoxic sediment zones. The OM-enriched sediment surface often needs higher oxygen demands and it causes a reduced oxygen penetration depth61, 62.
The long-term persistence of this event, in turn, causes the hypoxia stress on macrofauna assemblages and increases the risk of eutrophication 63, 64. In the intertidal areas, low-density
and the removal of large bioturbators (e.g., _M. liliana_, _M. stewartensis_ and _A. marina_) often lead to a lower consumption of carbon sourced from microphytobenthos (MPB) on sediment
surface65, 66. In our study, the shallow penetration of OM also indicates few labile OM resources (e.g., MPB) are transported to the sediment subsurface and incorporated to the nutrient
cycling. The composition of MPB shifts the trophic linkage between MPB and macrofauna breaks: macrofauna grazing on microphytes provides nutrients (e.g., ammoniacal nitrogen) to maintain the
MPB standing stock49. However, MP might break this linkage with increasing cyanobacteria biomass in the MPB community, which changes the nutrient cycling in the sediment45. The effect of MP
is concerning in these scenarios because the biogeochemical processes that rely on particle mixing by large macrofauna will need a longer time to recover, either as the toxicity of the
plastic decreases or as more tolerant species with similar functional traits replace the more sensitive species 67. Ecological consequences of MP are context-dependent on the species
composition and MP properties (e.g., concentrations); therefore, the outputs should be carefully interpreted due to the simplification of the model. Firstly, the parameter Db may be
sensitive to changes in the worm’s activities and deep particle mixing patterns, but it does not represent the changes on bivalve’s fluid oscillation. The hydraulic activities extend the
oxic zones and mediate the microbial activities periodically47, 68, 69, and this needs to be involved in the further assessment. Secondly, we have not included responses from microbial
community and microphytobenthos to MP. Previous studies have shown MP triggering microbial aggregation70, 71, shifting the microbial compositions 72 and increasing cyanobacteria biomass at
the sediment surface 45. While these effects may be most pronounced in shallow photic sediments, these are common in harbours and estuaries that often exhibit high MP concentrations. We
expect that excluding these components might deviate the prediction from the observation in a real-world experiment. For example, the growth of the cyanobacteria at the photic sediment
surface will reduce the downward diffusion of O2 fluxes, and O2 consumption decreases when gross photosynthesis increases73. In this circumstance, the observed O2 consumption rate from MP
treatments can be a net effect of increased aerobic respiration induced by OM accumulation and gross photosynthesis caused by cyanobacteria. Thirdly, the model assumption of complete
degradation of OM in a virtual semi-closed sediment zone34 leads to the analytical estimation of O2 consumption and N2 production via feedbacks to metabolic resource redistribution that is
tuned by parameter Db. Although N2 production in MP-treatments decreased by a small percentage owing to increased aerobic mineralization in worm and combined groups; a weakened nitrogen
cycling is likely a result of a chains-reaction when macrofauna loses its functional role in transporting labile OM and regulating microbial activities. This study has not included multiple
limiting factors (e.g., OM quality, NO3- concentration) in N-cycling, as well as the complexity of pathways of denitrification, including directly denitrifying NO3- in overlying water and
coupling of nitrification and denitrification processes at an oxic-anoxic sediment interface74. MP as synthetic polymers potentially participates in OM degradation75, and some materials
(PLA) can serve as carbon sources which stimulate both nitrification and denitrification rates72. In a eutrophic system, OM (quality and quantity) constrains denitrification when overlying
water provides sufficient NO3−; in a low-nutrient system, the NO3- supply from nitrification is the limiting factor for denitrification in the sediment74. In this case, we predicted that
when the bioturbation rate decreased because of MP, O2 demands increased because of OM accumulation. If this phenomenon co-occurs with decreased O2 penetration depth, the nitrification can
stop due to the limited O2 supply, and this decouples nitrification from denitrification in the sediment. These undefined relationships increase the difficulty in predicting MP’s impacts on
ecosystem functioning. The relationship between MP and bioturbation needs more empirical data to confirm before upscaling to a global-scale model76,77,78. CONCLUSION Our study highlights
that the impact of MP on bioturbation can result in broad-scale shifts in ecosystem functioning. These effects are the result of interaction between functional traits of bioturbating species
and concentration of MP. The strongest effects on function were driven by changes in the deep-burrowing behaviour of maldanid worms. This result indicates that substantial effects of MP
pollution on ecosystem function may be more evident in areas dominated by large deep burrowing species rather than in more degraded ecosystems dominated by other smaller macrofauna25, 49.
The differences in our estimates of Db from the maldanid worm and the tellinid bivalve highlight the importance of considering the specific mechanisms of bioturbation and how they can be
incorporated in biogeochemical models. Linking laboratory observation to the numerical model allows us to estimate the consequences at the ecosystem level of MP contamination. Future studies
can expand on both the range of functional traits of key species and consider community level effects as well as considering the hydraulic activities of macrofauna alongside particle
transport. METHODS MEASURING DB UNDER DIFFERENT MP LOADS SEDIMENT AND ANIMAL COLLECTION Sediment and animals, the tellinid bivalve _Macomona liliana_, and the maldanid polychaete
_Macroclymenella stewartensis,_ were collected during low tide from Whāngateau estuary (36°18′52.37" S, 174°46′17.09" E), New Zealand. Sediments (grain size: 164.94 ± 1.58 μm mean
± standard deviation, fine sand fraction: 55.5%, mud content:11.4%) were sieved through 500 μm mesh a to remove t macrofauna. Bivalve _M. liliana_ (minimum shell length: 3.8 cm, maximum
shell length: 4cm) and worm _M. stewartensis_ (body length: ~ 10 cm) were hand-collected from their habitats. The estimated wet weights for bivalve and worm at these sizes were 2.3 g and 0.2
g, respectively 40. MP PREPARATION Prewashed polypropylene plastic pellets (PP, diameter: 4 mm, LINGS limited, China) were frozen for 2 weeks at −80 °C to embrittle the PP particles. The
frozen raw materials were ground using a coffee mill (Coffee tech Limited, New Zealand). MP particles (hereafter: MP) were sieved through 500 μm mesh to control the size of MP (diameter <
500 μm). PREINCUBATION Before being combined with target species, MP were introduced to the top 1 cm surface sediment and incubated from 30th May to 17th, June, 2022. This step is designed
to reduce the resuspension of MP. A 2-week incubation allows microalgae to cover the surface of MP 79. This bio-stabilization (e.g., growth of biofilm and interact with microalgae) on the
sediment bed is the precondition for MP deposition in the sediment 6. Preserved MP and surface sediment were homogenized and incubated in individual containers (1cm depth, surface areas:
727.8 cm2). We created a gradient in MP concentrations with values of 0.02, 0.002, 0.0002 g cm−2 by surface areas of sediment. Sediments without plastic addition were incubated as a control.
Each control and MP treatment was replicated three times, with three blocks of sediment allocated in each container. These preincubated sediments were set up at temperature at ~ 16 °C, and
topped up with filtered, clean seawater. A gentle inflow rate ~ 35 ml min−1 was set to limit resuspension in the sediment columns. All MP treatments were randomly layout under four double
Aqua One Reflector Fluroglow T8 (40 W) sunlight tubes hung 55 cm above the water surface, and set on a 12 h light/dark cycle. The photosynthetic photon flux density (PAR, waveband 400–700
nm) on the surface of incubated water was measured by Li-Cor LI-190R quantum sensor coupled with a Li-Cor data-logger (Li-Cor, USA), with avg. 165.42 ± SD 5.60 μmol photons m−2 s−1. External
light was excluded by a blackout curtain. MAIN INCUBATION We measured the sediment reworking profiles from incubations with worms, bivalves, and a combination of the two in MP-contaminated
sediment. A total of 36 cylindric buckets (volume: 5 L, height: 24.5 cm) were submerged in the filtered water flow for at least 3 weeks to reduce the release of the plasticizers. All buckets
were filled with 11cm clean sieved-sediment and topped with 1 cm surface sediment (surface areas: 240.6 cm2) from the preincubation step. These sediments were settled for 24 h and placed in
a bath of sand-filtered seawater (salinity: 35.5, temperature: 16 °C, flow rate: ~ 80 ml min−1, water depth: 13 cm). Healthy worms and bivalves were placed on the surface sediment and
allowed to burrow into the sediment and acclimate for 24 h. The main incubation started with no worms or bivalves on the sediment surface. A total of 34 g of luminophores (florescence
painted natural sands, grain size diameter: 149.15 μm ± 0.51 mean ± standard deviation) were evenly spread through the water column to cover the surface sediment at a density of 0.14 g
luminophores cm−2 in the top 1 cm sediment. A control (0 g MP cm−2) and three MP concentration levels (g cm−2 surface areas of 1cm depth wet sediment) were used: (1) low concentration
(0.0002 g cm−2), (2) medium concentration (0.002 g cm−2) and (3) high concentration (0.02 g cm−2). These were crossed with three animal treatments (a) two _M. liliana_ (‘bivalve’), (b) two
_M. stewartensis_ (‘worm’) and (c) one _M. liliana_ with one _M. stewartensis_ (‘combined’). The density of two species in each treatment was 83.3 ind. m−2 and it is within the ranges of
natural density in the habitat 80. The two species naturally co-occur in New Zealand intertidal soft sediment40. These treatments were replicated three times and incubated from 18th June to
12th July in Leigh marine laboratory, The University of Auckland, New Zealand. Incubation buckets were randomly distributed under the same light condition as that in the preincubation step.
LUMINOPHORE—SEDIMENT SAMPLING Three sediment cores (10 cm depths, diameter: 2.7 cm) were collected from each bucket. At the end of incubation, the absence of luminophore tracers from the
surface sediment are the result of animal burrowing and feeding 81. Sediment cores were collected from these spots, and sliced into 2 cm increments as five subsamples and pooled from
individual buckets. LUMINOPHORE RECOVERY Subsamples were stirred for 1 h and digested by 15% H2O2 solution for 1 week in clean glass beakers to remove organic matter 82. The digested
subsamples were carefully rinsed through distilled water, placed in dust-free containers (70 ml), and freeze-dried for 72 h. Freeze-dried subsamples were weighed and homogenized. Visible
shell fragments were picked out from the containers. A cohesive tape (size: 4.0 cm × 4.2 cm, transparent) was vertically inserted to the container and vortexed for 60 s (800 rpm) to
homogenize all particles on the tape. The tape covered by luminophores and sediment particles was removed from the container and preserved in petri dishes, and the remaining sediment was
re-weighed. The difference on the sample weight was the weight of the particles that attached on the cohesive tape (g). Triplicated tape samples were extracted from each container. The
amount of the luminophores spreads through sediment section (0–2 cm, 2–4 cm, 4–6 cm, 6–8 cm and 8–10 cm depths) reflects the vertical sediment mixing by bioturbation for the luminophore
particle83, 84. Luminophore particles in the tape samples were photographed in a UV light chamber (34 × 26 × 30 cm), with four installed ultraviolet (UV) lights at top corners to deliver
consistent illumination. Tape samples were horizontally laid on the bench within the chamber, under a 12 cm distance from camera lens (48-mega pixels, 25× zoom-in). Sample photographs (3024
× 4032 pixels) were binarized as black and white (0: pixels in black areas, 1: pixels in florescent areas) and luminophore particles were calculated by Image J 1.53a (Plugins, Blob labeler).
The profiles of luminophore tracers from the laboratory test represents the sediment reworking by bioturbators interacting with MP (F24,180 = 39.037, p = 0.000, 3-way ANOVA test, See Table
S1, Supplementary); These observations were fitting to the deterministic bioturbation model (details as below). DERIVED DB BY FITTING SEDIMENT REWORKING PROFILES The observed profiles of
luminophore particles from the experiment were fitted to a deterministic bioturbation model to derived the bioturbation coefficients Db 77, 85. This method has been applied in previous
studies16, 81. The predicted luminophore transportation at each depth was calculated as: $$Lum\left( x \right) \, = \, \left( {\frac{{1}}{{\sqrt {\pi D_{b} t} }}} \right)e^{{ - x^{{2}}
/{4}D_{b} t}}$$ (1) where: $$\frac{\partial Lum(x)}{{\partial x}} = 0,\quad when\quad x = 0$$ $$Lum(x \to \infty , \, t) = 0;$$ Db is assumed to be consistent in time; t is the duration of
the experimental days, 21 days; x is the sediment layers 0–2, 2–4, 4–6, 6–8, 8–10 cm (nominal depths: 0, 2, 4, 6, 8 cm); Lum(x) is the luminophore particles at depth _x_; Db was derived from
a convergent iteration and the weighted regression of least-squares comparison between the observational (_obs_i_) and predicted luminophore particle (_pred_i_) profiles (see profile
example in Fig. 6) using the least square non-linear function (LSQNONLIN, Matlab, 2021b). The iteration continued until observational data has minimum distance to prediction, resulting in
the smallest residual values. $${\text{Residuals }} = \sum\nolimits_{i = 1}^{n} {\frac{{\left( {obs\_i - pred\_i} \right)^{2} }}{{obs_{i} + 1}}}$$ (2) Residual outputs have shown the fit of
the predicted values to the observational data (see Fig. S2). ESTIMATED IMPACTS ON ECOSYSTEM FUNCTIONS BY FITTING DB The simplified transport-reaction model34 was applied to evaluate the
ecosystem functioning associated to bioturbation in MP-contaminated system (parameters and constant values as shown in Table S2). We set a depth of 2 cm sediment as the boundary of oxic zone
for aerobic mineralization (Zo2) which is in line to a common range of redox potential layer in the sediment86. OM burial was considered negligible due to the dominance of bioturbation34.
The profile of OM concentration along sediment depth (C(x), mmol OM g−1): $$C\left( x \right) \, = \, C^{0} e^{{\left( { - x/z} \right)}} ;$$ (3) where Z is the effective zone of
bioturbation: $${\text{Z }} = \frac{{2D_{b} }}{{ - \omega + \sqrt {\omega^{2} + 4 D_{b} k}}};$$ (4) And the estimated OM concentration (C0, mmol OM g−1) initially received from
sediment–water interface (SWI) is: $$C^{0} = \frac{2F\_OM}{{\rho (1 - \phi )[\omega + \sqrt {\omega^{2} + 4D_{b} k]} }};$$ (5) The aerobic mineralization rate (R(oxic_Db), mmol OM cm−2
year−1) at oxic zone: $$R\left( {oxic\_D_{b} } \right) \, = \, Z_{o2} k*\rho (1 - \phi )*C_{avg} (D_{b} ) = Z_{o2} k\frac{F\_OM}{{D_{b} k}};$$ (6) where Cavg(Db) is the approximate average
OM concentration in the oxic zone when Db dominants the advective transport (ω = 0): $$C_{avg} \left( {D_{b} } \right) \, = \frac{F\_OM}{{\rho (1 - \phi )\sqrt {D_{b} k} }};$$ (7) The oxygen
consumption rates (mmol cm−2 year−1) for aerobic mineralization: $$F_{\_o2} \approx \, R\left( {oxic\_D_{b} } \right);$$ (8) The fraction of aerobic mineralization (β) accounting to OM
degradation: $$\beta = \frac{{R_{(oxic\_Db)} }}{F\_OM} = \frac{{Z_{O2} k\frac{{F_{OM} }}{{\sqrt {D_{b} k} }}}}{F\_OM} = Z_{O2} *\sqrt {\frac{k}{{D_{b} }}} ;$$ (9) The fraction of OM fluxes
for reduction processes that can enhance denitrification (R(N)) : $$R_{(N)} \cong F\_OM \, {-} \, R_{{\left( {oxic\_D_{b} } \right) \, }} = \, F\_OM \, * \, (1 - \beta )$$ (10) F_OM is a
fixed organic matter flux delivered to SWI as a boundary condition, average 0.6 mmol cm−2 year−1 in coastal environment; _k_ is the organic matter decay constant (year−1), k = 0.1; ω is the
advective velocity for solids and solutes, 0.1 cm year−1; ρ is the sediment density, 2.55 g cm−3; ϕ is the porosity, 0.35. DATA AVAILABILITY Supplementary contains all relevant data as
attached. REFERENCES * Law, K. L. & Thompson, R. C. Microplastics in the seas. _Science (80-)_ 345, 144–145 (2014). ADS CAS Google Scholar * Barnes, D. K. A., Galgani, F., Thompson,
R. C. & Barlaz, M. Accumulation and fragmentation of plastic debris in global environments. _Philos. Trans. R Soc. B Biol. Sci._ 364, 1985–1998 (2009). CAS Google Scholar * Chenillat,
F., Huck, T., Maes, C., Grima, N. & Blanke, B. Fate of floating plastic debris released along the coasts in a global ocean model. _Mar. Pollut. Bull._ 165, 112116 (2021). CAS PubMed
Google Scholar * Ockenden, A., Tremblay, L. A., Dikareva, N. & Simon, K. S. Towards more ecologically relevant investigations of the impacts of microplastic pollution in freshwater
ecosystems. _Sci. Total Environ._ 792, 148507 (2021). ADS CAS PubMed Google Scholar * Yokota, K. _et al._ Finding the missing piece of the aquatic plastic pollution puzzle: Interaction
between primary producers and microplastics. _Limnol. Oceanogr. Lett._ 2, 91–104 (2017). Google Scholar * Hope, J. A., Coco, G., Parsons, D. R. & Thrush, S. F. Microplastics interact
with benthic biostabilization processes. _Environ. Res. Lett._ 16, 124058 (2021). ADS CAS Google Scholar * Kane, I. A. _et al._ Seafloor microplastic hotspots controlled by deep-sea
circulation. _Science (80-)_ 368, 1140–1145 (2020). ADS CAS Google Scholar * Napper, I. E. & Thompson, R. C. Release of synthetic microplastic plastic fibres from domestic washing
machines: Effects of fabric type and washing conditions. _Mar. Pollut. Bull._ 112, 39–45 (2016). CAS PubMed Google Scholar * Turner, A. & Holmes, L. Occurrence, distribution and
characteristics of beached plastic production pellets on the island of Malta (central Mediterranean). _Mar. Pollut. Bull._ 62, 377–381 (2011). CAS PubMed Google Scholar * Wright, S. L.,
Rowe, D., Thompson, R. C. & Galloway, T. S. Microplastic ingestion decreases energy reserves in marine worms. _Curr. Biol._ 23, R1031–R1033 (2013). CAS PubMed Google Scholar * Green,
D. S., Boots, B., Sigwart, J., Jiang, S. & Rocha, C. Effects of conventional and biodegradable microplastics on a marine ecosystem engineer (_Arenicola marina_) and sediment nutrient
cycling. _Environ. Pollut._ 208, 426–434 (2016). CAS PubMed Google Scholar * You, Y., Thrush, S. F. & Hope, J. A. The impacts of polyethylene terephthalate microplastics (mPETs) on
ecosystem functionality in marine sediment. _Mar. Pollut. Bull._ 160, 111624 (2020). CAS PubMed Google Scholar * Urban-Malinga, B., Jakubowska, M. & Białowąs, M. Response of
sediment-dwelling bivalves to microplastics and its potential implications for benthic processes. _Sci. Total Environ._ 769, 144302 (2021). ADS CAS PubMed Google Scholar * Soetaert, K.
_et al._ Modeling 210Pb-derived mixing activity in ocean margin sediments: Diffusive versus nonlocal mixing. _J. Mar. Res._ 54, 1207–1227 (1996). Google Scholar * Meysman, F. J. R.,
Boudreau, B. P. & Middelburg, J. J. Relations between local, nonlocal, discrete and continuous models of bioturbation. _J. Mar. Res._ 61, 391–410 (2003). Google Scholar * Maire, O.,
Duchêne, J. C., Rosenberg, R., De Mendonça, J. B. & Grémare, A. Effects of food availability on sediment reworking in _Abra ovata_ and _A. nitida_. _Mar. Ecol. Prog. Ser._ 319, 135–153
(2006). ADS Google Scholar * Middelburg, J. J. Marine carbon biogeochemistry: A primer for earth system scientists. _Springer Briefs Earth Syst. Sci._
https://doi.org/10.1007/978-3-030-10822-9 (2019). Article Google Scholar * Michaud, E., Desrosiers, G., Mermillod-Blondin, F., Sundby, B. & Stora, G. The functional group approach to
bioturbation: The effects of biodiffusers and gallery-diffusers of the _Macoma balthica_ community on sediment oxygen uptake. _J. Exp. Mar. Biol. Ecol._ 326, 77–88 (2005). CAS Google
Scholar * Michaud, E., Desrosiers, G., Mermillod-Blondin, F., Sundby, B. & Stora, G. The functional group approach to bioturbation: II. The effects of the _Macoma balthica_ community on
fluxes of nutrients and dissolved organic carbon across the sediment-water interface. _J. Exp. Mar. Biol. Ecol._ 337, 178–189 (2006). CAS Google Scholar * Hillman, J. R., Lundquist, C.
J., Pilditch, C. A. & Thrush, S. F. The role of large macrofauna in mediating sediment erodibility across sedimentary habitats. _Limnol. Oceanogr._ 65, 683–693 (2020). ADS Google
Scholar * Shi, B. _et al._ Influence of macrobenthos (_Meretrix meretrix_ linnaeus) on erosion-accretion processes in intertidal flats: A case study from a cultivation zone. _J. Geophys.
Res. Biogeosci._ 125, 1–15 (2020). Google Scholar * Kristensen, E., Delefosse, M., Quintana, C. O., Flindt, M. R. & Valdemarsen, T. Influence of benthic macrofauna community shifts on
ecosystem functioning in shallow estuaries. _Front. Mar. Sci._ 1, 1–14 (2014). Google Scholar * Shull, D. H., Benoit, J. M., Wojcik, C. & Senning, J. R. Infaunal burrow ventilation and
pore-water transport in muddy sediments. _Estuar. Coast. Shelf Sci._ 83, 277–286 (2009). ADS CAS Google Scholar * Coppock, R. L. _et al._ Benthic fauna contribute to microplastic
sequestration in coastal sediments. _J. Hazard. Mater._ 415, 125583 (2021). CAS PubMed Google Scholar * Norkko, A., Villnäs, A., Norkko, J., Valanko, S. & Pilditch, C. Size matters:
Implications of the loss of large individuals for ecosystem function. _Sci. Rep._ 3, 1–8 (2013). Google Scholar * Gammal, J., Norkko, J., Pilditch, C. A. & Norkko, A. Coastal hypoxia
and the importance of benthic macrofauna communities for ecosystem functioning. _Estuar. Coasts_ 40, 457–468 (2017). CAS Google Scholar * Hillman, J. R., Lundquist, C. J., O’Meara, T. A.
& Thrush, S. F. loss of large animals differentially influences nutrient fluxes across a heterogeneous marine intertidal soft-sediment ecosystem. _Ecosystems_ 24, 272–283 (2020). Google
Scholar * Ladewig, S. M., Bianchi, T. S., Coco, G., Hope, J. A. & Thrush, S. F. A call to evaluate plastic’s impacts on marine benthic ecosystem interaction networks. _Environ. Pollut._
273, 116423 (2021). CAS PubMed Google Scholar * Hope, J. A., Coco, G., Parsons, D. M. & Thrush, S. F. Microplastics interact with benthic biostabilization processes. _J. Phys.
Energy_ 2, 1–31 (2020). Google Scholar * Van Prooijen, B. C., Montserrat, F. & Herman, P. M. J. A process-based model for erosion of _Macoma balthica_-affected mud beds. _Cont. Shelf
Res._ 31, 527–538 (2011). ADS Google Scholar * Gilbert, F., Stora, G. & Cuny, P. Functional response of an adapted subtidal macrobenthic community to an oil spill: macrobenthic
structure and bioturbation activity over time throughout an 18-month field experiment. _Environ. Sci. Pollut. Res._ 22, 15285–15293 (2015). CAS Google Scholar * Gong, Z. & Zhang, Q.
Impact of heavy metals (cadmium and copper) stress on the bioturbation potential of polychaete _Perinereis aibuhitensis_ (family Nereididae). _Mar. Environ. Res._ 177, 105621 (2022). CAS
PubMed Google Scholar * Boudreau, B. P. The mathematics of early diagenesis: From worms to waves. _Rev. Geophys._ 38, 389–416 (2000). ADS Google Scholar * Meysman, F. J. R., Galaktionov,
O. S., Madani, S. & Middelburg, J. J. Modelling biological interactions in aquatic sediments as coupled reactive transport. _Interact. Betw. Macro- Microorg. Mar. Sedim._
https://doi.org/10.1029/CE060p0359 (2013). Article Google Scholar * Dunn, S. M. & Aller, R. C. Macrofaunal mucus secretions and tube linings in marine sediments. _J. Mar. Res._ 5,
957–981 (2005). Google Scholar * Mermillod-Blondin, F., Rosenberg, R., François-Carcaillet, F., Norling, K. & Mauclaire, L. Influence of bioturbation by three benthic infaunal species
on microbial communities and biogeochemical processes in marine sediment. _Aquat. Microb. Ecol._ 36, 271–284 (2004). Google Scholar * Michaud, E., Aller, R. C. & Stora, G. Sedimentary
organic matter distributions, burrowing activity, and biogeochemical cycling: Natural patterns and experimental artifacts. _Estuar. Coast. Shelf Sci._ 90, 21–34 (2010). ADS CAS Google
Scholar * Herbert, R. A. Nitrogen cycling in coastal marine ecosystems. _FEMS Microbiol. Rev._ 23, 563–590 (1999). CAS PubMed Google Scholar * Joye, S. B. & Anderson, I. C. _Nitrogen
in the Marine Environment_. https://doi.org/10.1016/B978-0-12-372522-6.00019-0 (Elsevier Inc., 2008). * Schenone, S., O’Meara, T. A. & Thrush, S. F. Non-linear effects of macrofauna
functional trait interactions on biogeochemical fluxes in marine sediments change with environmental stress. _Mar. Ecol. Prog. Ser._ 624, 13–21 (2019). ADS CAS Google Scholar * Aller, R.
C. & Cochran, J. K. The critical role of bioturbation for particle dynamics, priming potential, and organic c remineralization in marine sediments: Local and basin scales. _Front. Earth
Sci._ 7, 1–14 (2019). Google Scholar * Pischedda, L., Poggiale, J. C., Cuny, P. & Gilbert, F. Imaging oxygen distribution in marine sediments. The importance of bioturbation and
sediment heterogeneity. _Acta Biotheor._ 56, 123–135 (2008). CAS PubMed Google Scholar * Welsh, D. T. It’s a dirty job but someone has to do it: The role of marine benthic macrofauna in
organic matter turnover and nutrient recycling to the water column. _Chem. Ecol._ 19, 321–342 (2003). CAS Google Scholar * Townsend, M., Thrush, S. F., Hewitt, J. E., Lohrer, A. M. &
McCartain, L. Behavioural changes in the Tellinid bivalve _Macomona liliana_ (Iredale, 1915) following exposure to a thin terrigenous sediment deposition event: Evidence from time-lapse
photography. _Cah. Biol. Mar._ 55, 475–483 (2014). Google Scholar * Hope, J. A., Coco, G. & Thrush, S. F. effects of polyester microfibers on microphytobenthos and sediment-dwelling
infauna. _Environ. Sci. Technol._ https://doi.org/10.1021/acs.est.0c00514 (2020). Article PubMed Google Scholar * Thrush, S. F. _et al._ Cumulative stressors reduce the self-regulating
capacity of coastal ecosystems. _Ecol. Appl._ 31, e02223 (2021). PubMed Google Scholar * Volkenborn, N. _et al._ Intermittent bioirrigation and oxygen dynamics in permeable sediments: An
experimental and modeling study of three tellinid bivalves. _J. Mar. Res._ 70, 794–823 (2012). CAS Google Scholar * Mazik, K. & Elliott, M. The effects of chemical pollution on the
bioturbation potential of estuarine intertidal mudflats. _Helgol. Mar. Res._ 54, 99–109 (2000). ADS Google Scholar * Thrush, S. F., Hewitt, J. E., Gibbs, M., Lundquist, C. & Norkko, A.
Functional role of large organisms in intertidal communities: Community effects and ecosystem function. _Ecosystems_ 9, 1029–1040 (2006). Google Scholar * Kauppi, L. _et al._ Changes in
macrofauna bioturbation during repeated heatwaves mediate changes in biogeochemical cycling of nutrients. _Front. Mar. Sci._ 9, 1–13 (2023). Google Scholar * Vlaminck, E., Cepeda, E.,
Moens, T. & Van Colen, C. Ocean acidification modifies behaviour of shelf seabed macrofauna: A laboratory study on two ecosystem engineers, _Abra alba_ and _Lanice conchilega_. _J. Exp.
Mar. Biol. Ecol._ 558, 151831 (2023). Google Scholar * Siwicka, E., Thrush, S. F. & Hewitt, J. E. Linking changes in species–trait relationships and ecosystem function using a network
analysis of traits. _Ecol. Appl._ 30, 1–10 (2020). Google Scholar * Schenone, S. & Thrush, S. F. Unraveling ecosystem functioning in intertidal soft sediments: the role of
density-driven interactions. _Sci. Rep._ 10, 1–9 (2020). Google Scholar * Hope, J. A., Coco, G., Ladewig, S. M. & Thrush, S. F. The distribution and ecological effects of microplastics
in an estuarine ecosystem. _Environ. Pollut._ 288, 117731 (2021). CAS PubMed Google Scholar * Zhou, Z. _et al._ Vertical microplastic distribution in sediments of Fuhe River estuary to
Baiyangdian Wetland in Northern China. _Chemosphere_ 280, 130800 (2021). ADS CAS PubMed Google Scholar * Bermúdez, M. _et al._ Unravelling spatio-temporal patterns of suspended
microplastic concentration in the Natura 2000 Guadalquivir estuary (SW Spain): Observations and model simulations. _Mar. Pollut. Bull._ 170, 112622 (2021). PubMed Google Scholar * Chen,
Z., Bowen, M., Li, G., Coco, G. & Hall, B. Retention and dispersion of buoyant plastic debris in a well-mixed estuary from drifter observations. _Mar. Pollut. Bull._ 180, 113793 (2022).
CAS PubMed Google Scholar * Sadri, S. S. & Thompson, R. C. On the quantity and composition of floating plastic debris entering and leaving the Tamar Estuary, Southwest England. _Mar.
Pollut. Bull._ 81, 55–60 (2014). CAS PubMed Google Scholar * Carson, H. S., Colbert, S. L., Kaylor, M. J. & McDermid, K. J. Small plastic debris changes water movement and heat
transfer through beach sediments. _Mar. Pollut. Bull._ 62, 1708–1713 (2011). CAS PubMed Google Scholar * Ladewig, S. M., Coco, G., Hope, J. A., Vieillard, A. M. & Thrush, S. F.
Real-world impacts of microplastic pollution on sea floor ecosystem function. _Sci. Total Environ._ 858, 160114 (2023). ADS CAS PubMed Google Scholar * Rosenberg, R., Nilsson, H. C.
& Diaz, R. J. Response of benthic fauna and changing sediment redox profiles over a hypoxic gradient. _Estuar. Coast. Shelf Sci._ 53, 343–350 (2001). ADS CAS Google Scholar * Yu, L.
_et al._ Modeling the role of riverine organic matter in hypoxia formation within the coastal transition zone off the Pearl River Estuary. _Limnol. Oceanogr._ 66, 452–468 (2021). ADS CAS
Google Scholar * Morris, J. T. & Bradley, P. M. Effects of nutrient loading on the carbon balance of coastal wetland sediments. _Limnol. Oceanogr._ 44, 699–702 (1999). ADS CAS Google
Scholar * Paerl, H. W. Assessing and managing nutrient-enhanced eutrophication in estuarine and coastal waters: Interactive effects of human and climatic perturbations. _Ecol. Eng._ 26,
40–54 (2006). Google Scholar * Schenone, S. _et al._ Mapping the delivery of ecological functions combining field collected data and unmanned aerial vehicles (UAVs). _Ecosystems_
https://doi.org/10.1007/s10021-021-00694-w (2021). Article Google Scholar * Volkenborn, N., Hedtkamp, S. I. C., van Beusekom, J. E. E. & Reise, K. Effects of bioturbation and
bioirrigation by lugworms (_Arenicola marina_) on physical and chemical sediment properties and implications for intertidal habitat succession. _Estuar. Coast. Shelf Sci._ 74, 331–343
(2007). ADS Google Scholar * Middelburg, J. J. & Levin, L. A. Coastal hypoxia and sediment biogeochemistry. _Biogeosciences_ 6, 1273–1293 (2009). ADS CAS Google Scholar *
Volkenborn, N., Polerecky, L., Wethey, D. S., DeWitt, T. H. & Woodin, S. A. Hydraulic activities by ghost shrimp _Neotrypaea californiensis_ induce oxic-anoxic oscillations in sediments.
_Mar. Ecol. Prog. Ser._ 455, 141–156 (2012). ADS CAS Google Scholar * Woodin, S. A. _et al._ Same pattern, different mechanism: Locking onto the role of key species in seafloor ecosystem
process. _Sci. Rep._ 6, 1–11 (2016). Google Scholar * Michels, J., Stippkugel, A., Lenz, M., Wirtz, K. & Engel, A. Rapid aggregation of biofilm-covered microplastics with marine
biogenic particles. _Proc. R. Soc. B Biol. Sci._ 285, 20181203 (2018). Google Scholar * Rogers, K. L., Carreres-Calabuig, J. A., Gorokhova, E. & Posth, N. R. Micro-by-micro
interactions: How microorganisms influence the fate of marine microplastics. _Limnol. Oceanogr. Lett._ 5, 18–36 (2020). CAS Google Scholar * Seeley, M. E., Song, B., Passie, R. & Hale,
R. C. Microplastics affect sedimentary microbial communities and nitrogen cycling. _Nat. Commun._ 11, 1–10 (2020). Google Scholar * Glud, R. N. Oxygen dynamics of marine sediments. _Mar.
Biol. Res._ 4, 243–289 (2008). Google Scholar * Vieillard, A. M. & Thrush, S. F. Ecogeochemistry and denitrification in non-eutrophic coastal sediments. _Estuar. Coasts_ 44, 1866–1882
(2021). CAS Google Scholar * Shang, H. A generic hierarchical model of organic matter degradation and preservation in aquatic systems. _Commun. Earth Environ._ 4, 1–10 (2023). ADS Google
Scholar * Archer, D. E., Morford, J. L. & Emerson, S. R. A model of suboxic sedimentary diagenesis suitable for automatic tuning and gridded global domains. _Global Biogeochem. Cycles_
16, 171–1721 (2002). Google Scholar * Boudreau, B. P. _Diagenetic Models and Their Implementation: Modelling Transport and Reactions in Aquatic Sediments_ (Springer, 1996). Google Scholar
* Middelburg, J. J., Soetaert, K. & Herman, P. M. J. Empirical relationships for use in global diagenetic models. _Deep. Res. Part I Oceanogr. Res. Pap._ 44, 327–344 (1997). ADS CAS
Google Scholar * Fazey, F. M. C. & Ryan, P. G. Biofouling on buoyant marine plastics: An experimental study into the effect of size on surface longevity. _Environ. Pollut._ 210, 354–360
(2016). CAS PubMed Google Scholar * Hailes, S. F. & Hewitt, J. E. _Manukau Harbour Ecological Monitoring Programme : Report on Data Collected up Until February 2007_. Vol. 4525
(2007). * Maire, O., Duchêne, J. C., Bigot, L. & Grémare, A. Linking feeding activity and sediment reworking in the deposit-feeding bivalve _Abra ovata_ with image analysis, laser
telemetry, and luminophore tracers. _Mar. Ecol. Prog. Ser._ 351, 139–150 (2007). ADS Google Scholar * Masura, J., Baker, J., Foster, G. & Arthur, C. _Laboratory Methods for the
Analysis of Microplastics in the Marine Environment: Recommendations for Quantifying Synthetic Particles in Waters and Sediments_. (NOAA Technical Memorandum NOS-OR&R-48, 2015). *
Bernard, G. _et al._ Experimental assessment of the effects of temperature and food availability on particle mixing by the bivalve abra alba using new image analysis techniques. _PLoS One_
11, 1–23 (2016). Google Scholar * Gerino, M. _et al._ Comparison of different tracers and methods used to quantify bioturbation during a spring bloom: 234-thorium, luminophores and
chlorophyll a. _Estuar. Coast. Shelf Sci._ 46, 531–547 (1998). ADS Google Scholar * Crank, J. _The Mathematics of Diffusion_ (Oxford University Press, 1975). MATH Google Scholar * Van
Kessel, J. F. The relation between redox potential and denitrification in a water-sediment system. _Water Res._ 12, 285–290 (1978). Google Scholar Download references ACKNOWLEDGEMENTS We
thank Ocean Change II project for research support. We appreciate the support from seafloor ecology team and staff in Leigh Marine laboratory, the University of Auckland. AUTHOR INFORMATION
AUTHORS AND AFFILIATIONS * Institute of Marine Science, The University of Auckland, Auckland, 1010, New Zealand Yuxi You, Alice Della Penna & Simon Francis Thrush * School of Biology
Science, The University of Auckland, Auckland, 1010, New Zealand Alice Della Penna Authors * Yuxi You View author publications You can also search for this author inPubMed Google Scholar *
Alice Della Penna View author publications You can also search for this author inPubMed Google Scholar * Simon Francis Thrush View author publications You can also search for this author
inPubMed Google Scholar CONTRIBUTIONS Y.Y. led the project, designed and ran the experiment and the laboratory analysis and analysed the data. S.F.T. and A.D.P. supervised the project and
provided consultancy for experimental design and statistical analysis. Y.Y. led the writing of the manuscript. S.F.T. and A.D.P. contributed to the writing and the revision of the
manuscript. CORRESPONDING AUTHOR Correspondence to Yuxi You. ETHICS DECLARATIONS COMPETING INTERESTS The authors declare no competing interests. ADDITIONAL INFORMATION PUBLISHER'S NOTE
Springer Nature remains neutral with regard to jurisdictional claims in published maps and institutional affiliations. SUPPLEMENTARY INFORMATION SUPPLEMENTARY INFORMATION. RIGHTS AND
PERMISSIONS OPEN ACCESS This article is licensed under a Creative Commons Attribution 4.0 International License, which permits use, sharing, adaptation, distribution and reproduction in any
medium or format, as long as you give appropriate credit to the original author(s) and the source, provide a link to the Creative Commons licence, and indicate if changes were made. The
images or other third party material in this article are included in the article's Creative Commons licence, unless indicated otherwise in a credit line to the material. If material is
not included in the article's Creative Commons licence and your intended use is not permitted by statutory regulation or exceeds the permitted use, you will need to obtain permission
directly from the copyright holder. To view a copy of this licence, visit http://creativecommons.org/licenses/by/4.0/. Reprints and permissions ABOUT THIS ARTICLE CITE THIS ARTICLE You, Y.,
Della Penna, A. & Thrush, S.F. Modelled broad-scale shifts on seafloor ecosystem functioning due to microplastic impacts on bioturbation. _Sci Rep_ 13, 17121 (2023).
https://doi.org/10.1038/s41598-023-44425-8 Download citation * Received: 16 June 2023 * Accepted: 08 October 2023 * Published: 10 October 2023 * DOI:
https://doi.org/10.1038/s41598-023-44425-8 SHARE THIS ARTICLE Anyone you share the following link with will be able to read this content: Get shareable link Sorry, a shareable link is not
currently available for this article. Copy to clipboard Provided by the Springer Nature SharedIt content-sharing initiative