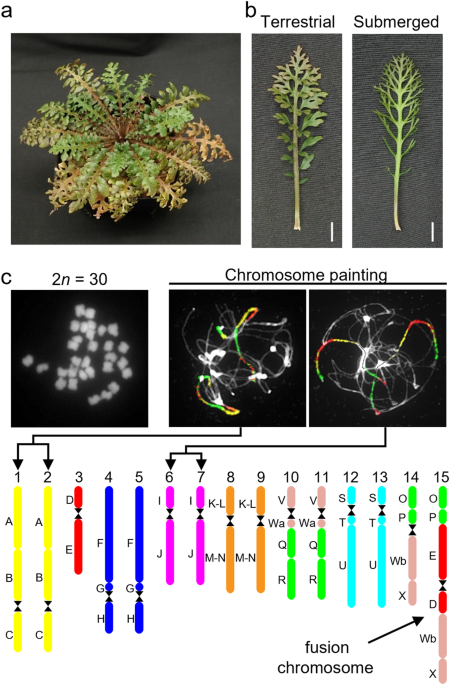
A chromosome-level genome assembly for the amphibious plant rorippa aquatica reveals its allotetraploid origin and mechanisms of heterophylly upon submergence
- Select a language for the TTS:
- UK English Female
- UK English Male
- US English Female
- US English Male
- Australian Female
- Australian Male
- Language selected: (auto detect) - EN
Play all audios:

ABSTRACT The ability to respond to varying environments is crucial for sessile organisms such as plants. The amphibious plant _Rorippa aquatica_ exhibits a striking type of phenotypic
plasticity known as heterophylly, a phenomenon in which leaf form is altered in response to environmental factors. However, the underlying molecular mechanisms of heterophylly are yet to be
fully understood. To uncover the genetic basis and analyze the evolutionary processes driving heterophylly in _R. aquatica_, we assembled the chromosome-level genome of the species.
Comparative chromosome painting and chromosomal genomics revealed that allopolyploidization and subsequent post-polyploid descending dysploidy occurred during the speciation of _R.
aquatica_. Based on the obtained genomic data, the transcriptome analyses revealed that ethylene signaling plays a central role in regulating heterophylly under submerged conditions, with
blue light signaling acting as an attenuator of ethylene signal. The assembled _R. aquatica_ reference genome provides insights into the molecular mechanisms and evolution of heterophylly.
SIMILAR CONTENT BEING VIEWED BY OTHERS STRUCTURE AND EVOLUTION OF THE FORSYTHIEAE GENOME ELUCIDATED BY CHROMOSOME-LEVEL GENOME COMPARISON OF _ABELIOPHYLLUM DISTICHUM_ AND _FORSYTHIA OVATA_
(OLEACEAE) Article Open access 18 February 2025 A GENOME ASSEMBLY FOR _ORINUS KOKONORICA_ PROVIDES INSIGHTS INTO THE ORIGIN, ADAPTIVE EVOLUTION AND FURTHER DIVERSIFICATION OF TWO CLOSELY
RELATED GRASS GENERA Article Open access 02 December 2023 CHROMOSOME-LEVEL GENOME ASSEMBLY OF A DESTRUCTIVE LEAF-MINING MOTH _ERIOCRANIA SEMIPURPURELLA ALPINA_ Article Open access 02 January
2025 INTRODUCTION Land plants evolved from aquatic green algae approximately 450 million years ago. Some land plants re-entered and adapted to aquatic environments and are called aquatic
plants. Aquatic plants, also known as hydrophytes or macrophytes, evolved various traits to survive in aquatic habitats, such as narrow and flexible leaf blades for efficient gas exchange,
aerenchyma formation, and a high capacity for vegetative reproduction. Among aquatic plants, there exists a group referred to as amphibious plants. These plants are primarily found in
environments characterized by fluctuating water levels, such as at the edge of water, and are capable of thriving both in terrestrial and submerged conditions. To adapt to these fluctuating
environments, phenotypic plasticity plays a crucial role in amphibious plants. This is particularly evident in the phenomenon called heterophylly, in which a marked change occurs in leaf
shape and function between terrestrial and submerged conditions1,2. Submerged leaves are often more finely dissected and narrower than terrestrial leaves3,4, and stomatal formation is
suppressed underwater5,6,7, allowing for efficient photosynthesis in both aquatic and terrestrial environments. Studying amphibious plants can provide valuable insight into the mechanisms of
adaptation and evolutionary processes of plants in aquatic environments. The adaptive traits of amphibious plants have long been intriguing botanists1,8,9,10,11. Several new models species,
such as _Rorippa aquatica_ (tribe Cardamineae, Brassicaceae)3, _Hygrophila difformis_ (Acanthaceae)4,12, _Ranunculus trichophyllus_ (Ranunculaceae)6, and _Callitriche palustris_
(Callitricheae)13 were developed for studying amphibious plant adaptations. However, despite extensive physiological and morphological studies on this topic1,8,9,10,11, the molecular
mechanisms remain largely enigmatic, possibly due to a lack of genomic information for these model amphibious plants. _Rorippa aquatica_, an amphibious plant found in North American bays of
lakes, ponds, and streams14, exhibits dramatic heterophylly in response to various environmental cues, such as temperature, light quantity, and submergence3. The leaves of this species
become more deeply dissected and narrower underwater than in the air (Fig. 1a, b). An earlier study demonstrated the mechanism of heterophylly in response to temperature in _R. aquatica_.
Changes in the expression of the _KNOTTED1-LIKE HOMEOBOX_ (_KNOX1_) gene in response to temperature and light intensity led to altered concentrations of gibberellins and cytokinin in leaf
primordia, which in turn alters leaf morphology3. We have previously reported that the somatic cells of _R. aquatica_ have 30 chromosomes15, whereas the base chromosome number (x) in related
Cardamineae species is eight and diploid species have 2_n_ = 2x = 1616, suggesting that _R. aquatica_ has a polyploid origin. Furthermore, the fact that the chromosome number of _R.
aquatica_ is not a multiple of eight indicates that its genome was restructured after polyploidization. In addition to exhibiting remarkable heterophylly, _R. aquatica_ propagates
vegetatively in nature. It produces flowers with white petals from April to August in its natural habitat17, and inducing floral differentiation under low-temperature conditions can be
achieved in laboratory settings18. The rarity of seed production may be caused by self-incompatibility17, which leads to the formation of self-clonal colonies. This species can regenerate
from detached leaf fragments and is a subject of research in the field of plant regeneration19. Furthermore, _R. aquatica_ is a member of the Brassicaceae and is closely related to
_Arabidopsis thaliana_ and _Cardamine hirsuta_ (tribe Cardamineae, Brassicaceae), a model plant for compound leaf development20,21,22, making it suitable as an experimental material.
Therefore, if genomic information on _R. aquatica_ is revealed, it will not only contribute to the elucidation of the molecular mechanisms and evolutionary basis of adaptation of amphibious
plants, but also to breeding studies on improving flood tolerance in Brassicaceae crops (cabbage, turnip, rapeseed, etc.). In the present study, we assembled the genome of _R. aquatica_ at
the chromosome level, revealed its chromosome architecture, and reconstructed its origin and evolution. This is the first study in which genomic information was completed at the chromosome
level for an amphibious plant that exhibits remarkable heterophylly. In addition, we performed the transcriptome analysis using genomic information to reveal the mechanisms of heterophylly
in response to submergence. Our results may shed light on the molecular basis of amphibious plant adaptations to fluctuating environments. RESULTS CHROMOSOME ARCHITECTURE OF _RORIPPA
AQUATICA_ REVEALED VIA COMPARATIVE CYTOGENOMICS _R. aquatica_ has 15 chromosome pairs (2_n_ = 30, hereafter listed as chromosomes RaChr01 to RaChr15)15 (Fig. 1c). We examined the _R.
aquatica_ genome structure and evolutionary processes via comparative chromosome painting (CCP) based on the localization of contigs of chromosome-specific Bacterial Artificial Chromosome
(BAC) clones of _A. thaliana_ on meiotic (pachytene) chromosomes (see Fig. 1c for examples of CCP). The painting probes were designed to reflect the system of 22 ancestral genomic blocks
(GBs, labeled as A to X)23,24 and eight chromosomes of the ancestral Cardamineae genome25. As all 22 GBs were found to be duplicated within the _R. aquatica_ haploid chromosome complement,
the species has a tetraploid origin (Fig. 1c). We used CCP to reconstruct a complete comparative cytogenetic map of _R. aquatica_ (Fig. 1c) and compared it to that of the ancestral genome of
the tribe Cardamineae with eight chromosomes25. Fourteen of the 15 chromosome pairs in _R. aquatica_ (RaChr01–RaChr14) are shared with the ancestral Cardamineae genome. Due to its polyploid
origin, the _R. aquatica_ genome contains six pairs of Cardamineae homeologues: AK1 (GBs A + B + C; RaChr01 and RaChr02), AK3 (F + G + H; RaChr04 and RaChr05), AK4 (I + J; RaChr06 and
RaChr07), AK5 [(K–L) + (M–N); RaChr08 and RaChr09], AK6/8 (V+Wa+Q + R; RaChr10 and RaChr11), and AK7 (S + T + U; RaChr12 and RaChr13). Chromosomes RaChr03 and RaChr14 are homeologous to
ancestral chromosomes AK2 (D + E) and AK8/6 (O + P+Wb+X), respectively. Chromosome RaChr15 (O + P + E + D+Wb+X) originated via nested chromosome insertion (NCI) of the AK2 homeologue into
the centromere of the AK8/6 homeologue. At least three paracentric inversions post-dated the NCI event (Fig. 2). Comparative cytogenomic analysis confirmed the tetraploid origin of _R.
aquatica_ and allowed us to reconstruct the structure of the RaChr15 fusion chromosome. CHROMOSOMAL GENOME ASSEMBLY AND ANNOTATION We assembled the _R. aquatica_ genome and predicted its
gene structure. As reference, we used _R. aquatica_ accession N, for which the heterophylly was highly responsive to temperature18. Hybrid genome assembly with Illumina short reads and
PacBio long reads was performed using the MaSuRCA assembler26. The assembled draft contigs were scaffolded using Hi-C Seq reads. Hi-C Seq provides information about the physical contact
between the genomic loci in the nuclei. Therefore, given that the chromosomes are clustered in the nuclei, Hi-C Seq data scaffolding allows chromosome-level assembly. This generated 15
chromosome-level sequences and 2040 fragments (Supplementary Table 1). The total length of the chromosome sequences and whole sequences were approximately 414 and 452 Mb, respectively,
similar to that of the expected genome size (420–450 Mb) obtained using k-mer counting (Supplementary Fig. 1 and Supplementary Table 2). Benchmarking Universal Single-Copy Orthologs (BUSCO)
was used to evaluate the assembled genome quality (Supplementary Fig. 2). In total, 1614 conserved single-copy land plant genes were screened within the _R. aquatica_ genome, and 99.1% were
identified, indicating the high reliability of the assembled genome. Among the 1614 genes, 57.3% of the genes were found to be duplicated. Repeat sequences in the genome were identified
(Supplementary Table 3) and masked for subsequent analyses. Gene structures were predicted using the Program to Assemble Spliced Alignments (PASA) pipeline27,28. The prediction results
obtained using several methods were merged into an integrated gene structure, resulting in the identification of 46,197 genes (Supplementary Table 4). BUSCO assessment to protein sequences
from _R. aquatica_ gene data identified 94.4% of the conserved genes (Supplementary Fig. 2), indicating the high reliability of the gene annotation. _R. aquatica_ has 1.6–1.7 times the
number of genes than that of diploid Brassicaceae species such as _A. thaliana_ (27,416 genes) and _C. hirsuta_ (29,458 genes). EVOLUTIONARY PROCESSES REVEALED VIA COMPARATIVE GENOMICS
Although the ancestral chromosome number of Cardamineae is x = 8, _R. aquatica_ has a hypotetraploid chromosome number (2_n_ = 30). This chromosome number and the structure of the _R.
aquatica_ genome were elucidated by cytogenomic analyses (Figs. 1c and 2) as a whole-genome duplication followed by a chromosome fusion that reduced the 16 chromosome pairs to 15. To further
explore this evolutionary process in _R. aquatica_, a comparative genomics analysis was conducted based on the chromosome sequences. Using OrthoFinder analysis of the entire genome, a
genome-level phylogeny of _R. aquatica_ and related species was constructed. The longest protein sequences of each gene were extracted and used as the genome-level protein dataset in the
present analysis. The datasets of 24 species were obtained from public genome databases. For intra-genus analyses, the preliminary dataset of _Rorippa islandica_ (2_n_ = 16)29 was prepared
by genome assembly, and subsequent gene prediction using public genome-seq read data. Based on the constructed phylogenetic tree, _R. aquatica_ and _R. islandica_ were placed in the clade
that includes _Arabidopsis_ (Supplementary Fig. 3), which corresponded to the Brassicaceae clade A30. _Barbarea vulgaris_ is the closest species, and _C. hirsuta_ is the more immediate
sister of the genus _Rorippa_, which coincide with past phylogenetic study with some nuclear genes31. To investigate the origin of the _R. aquatica_ genome, we compared the chromosome-level
assemblies of _R. aquatica_ and _C. hirsuta_ (Supplementary Fig. 4). Multiple alignments based on nucleotide similarity showed that each chromosome of _C. hirsuta_ was similar to two _R.
aquatica_ chromosomes, indicating a tetraploid origin of the latter species. Furthermore, the collinearity of the RaChr15 fusion chromosome with the _C. hirsuta_ chromosomes Chr2 and Chr8
indicated that this _R. aquatica_ chromosome was formed via an NCI involving the _Rorippa_ homeologues RaChr03 and RaChr14, which is consistent with our CCP-based results (Fig. 2). The
divergence ages of duplicated genes could indicate when _R. aquatica_ attained its tetraploid-like characteristics. To estimate divergence ages in the Brassicaceae, we selected eight species
(_A. thaliana_, _A. lyrata_, _Barbarea vulgaris_, _Capsella rubella_, _Cardamine hirsuta_, _Eutrema salsugineum_, _R. aquatica_, and _R. islandica_). All genes in each genome were clustered
into orthogroups (i.e., groups consisting of orthologous genes) based on protein similarity (Supplementary Data 1). In total, 10,868 single-copy orthogroups were found by comparing six of
the eight species (excluding _R. aquatica_ and _R. islandica_). In _R. islandica_, almost all (91.5%) were single-copy genes, whereas in _R. aquatica_, 58.6% were duplicated genes
(Supplementary Table 5). This suggests that the _R. aquatica_ genome underwent large-scale gene duplication. To estimate species divergence and gene duplication ages, we calculated the
synonymous nucleotide substitution rate (Ks) between gene orthologs, both inter- and intra-species. To calculate Ks, we used the longest coding DNA sequences of each gene conserved as a
single copy in the seven Brassicaceae species and duplicated in _R. aquatica_; this resulted in 5909 sequences. The Ks distributions of _R. aquatica_ compared with those of other species and
_R. islandica_ were similar, reflecting phylogenetic relatedness (Supplementary Fig. 5). Divergence ages were estimated using Ks as T (in years) = Ks/(2 * _μ_), where _μ_ = 6.51648E−09
synonymous substitutions/site/year for Brassicaceae32. Based on this result, the divergence times between _Cardamine_ and _Rorippa_ and between _Barbarea_ and _Rorippa_ are 13.7–14.2 million
years ago (Mya) and 10.5–10.8 Mya, respectively (Supplementary Table 6). The Ks distribution based on the duplicated paralogs of _R. aquatica_ has a single peak with a median of Ks = 0.102,
which corresponds to a divergence of 7.8 Mya. This suggests that large-scale gene duplication in _R. aquatica_ occurred after the _Barbarea_/_Rorippa_ divergence and most likely occurred at
the whole-genome level. In _R. aquatica_ and _R. islandica_, the median of Ks is 0.073, corresponding to a divergence of 5.6 Mya. Although _R. islandica_ has diploid characteristics
(Supplementary Table 5) and a chromosome number of 2_n_ = 1629, our findings indicate that these two _Rorippa_ species diverged after the whole-genome duplication (WGD). Ks between _R.
aquatica_ and _R. islandica_ were analyzed at the chromosome level to elucidate this conflict. The chromosomes of _R. aquatica_ form two subgenome groups based on their distribution of the
calculated Ks relative to that of the _R. islandica_ genome (Fig. 3a and Supplementary Tables 7, 8) and they seem to be located in same phylogenetic distances for _B. vulgaris_ and _C.
hirsuta_ due to no clear segregation based on their Ks (Supplementary Fig. 6). The first group, named subgenome A, includes eight chromosomes (RaChr01, -03, -05, -07, -08, -10, -13, and -14)
with a median Ks value of approximately 0.05. These chromosomes are closer to those of _R. islandica_. Subgenome B includes seven chromosomes (RaChr02, -04, -06, -09, -11, -12, and -15)
with a median Ks value of approximately 0.09. These chromosomes are phylogenetically more distant from those of _R. islandica_; their Ks values relative to _R. islandica_ are similar to
those between the duplicated _R. aquatica_ genes. The fact that _R. aquatica_ has two subgenomes with different divergence ages indicates an allotetraploid origin caused by hybridization
between two ancestral _Rorippa_ species. Integration of various genomic analyses clarified the structure of the _R. aquatica_ genome (Fig. 3b). The homologous chromosomes in each subgenome
show a similar distribution of genes and long terminal repeats (LTRs). The peaks of the LTR distribution in each chromosome indicate centromeric regions. These results also suggest that gene
duplication in the species occurred via a WGD. Comparative genomics revealed the evolutionary process of establishing the present _R. aquatica_ (Fig. 3b): assuming an allotetraploid origin
of _R. aquatica_, _Rorippa_ split into the subgenome groups A and B approx. 7.2 Mya (Ks = 0.09). In the subgenome A group, divergence into the ancestor of _R. islandica_ and the parental
species of _R. aquatica_ occurred at 4.2 Mya (Ks = 0.05). Subsequent hybridization of two species from different subgenome groups resulted in the formation of the allotetraploid origin of
_R. aquatica_. Phylogenetic analysis based on plastid sequences placed _R. islandica_ in a clade distant from _R. aquatica_15. This suggests a paternal origin of subgenome A of _R.
aquatica_. Our analysis does not identify the seed parent of subgenome B. Based on Ks analysis, the fusion chromosome RaChr15 was formed by intra-subgenomic fusion. The median Ks value of
RaChr15 versus _R. islandica_ was approximately 0.09, which is similar to that of the other chromosomes of subgenome B (Fig. 3a and Supplementary Table 7). Chromosomes RaChr03 and RaChr14,
within subgenome A, remained as independent chromosomes. TRANSCRIPTOME ANALYSIS REVEALS THE PATHWAY UNDERLYING HETEROPHYLLY IN RESPONSE TO SUBMERGENCE We conducted RNA-seq gene expression
analysis using the assembled _R. aquatica_ genome data to elucidate the mechanism of heterophylly in response to submergence (information about RNA-seq read data of all transcriptome
analyses is shown in Supplementary Data 2). First, we observed the morphology of young leaves over time to determine the timing of leaf shape change upon submergence (Fig. 4a). After one day
of submergence, submerged and terrestrial leaves did not differ morphologically. After four days of submergence, the young-leaf margin serrations became deeper in the submerged than in the
terrestrial plants. After 7 days of submergence, leaf incisions were significantly deeper in the submerged leaves. To reveal the gene expression patterns of early response to submergence as
well as the early stage of leaf morphology differentiation, shoot apices containing young leaves were sampled at 1 h and four days after submergence and RNA-seq analyses were performed. The
RNA-seq reads for a 1 h submergence treatment obtained in an earlier study (DRA014113)33 were incorporated into the analyses. As a result of identification of differentially expressed genes
(DEGs) with certain threshold (FDR < 0.01 and |Log2FC| > 1), we identified 787 upregulated and 1091 downregulated genes 1 h after submergence, which increased to 5358 upregulated and
4945 downregulated genes after 4 days of submergence (Fig. 4b). The submergence-responsive DEGs were classified into three classes according to the timing of expression. The genes whose
expression changed only within 1 h of submergence were classified as “early response genes.” The genes whose expression changed after 1 h as well as after 4 days of submergence were
classified as “throughout response genes.” The genes whose expression changed only after 4 days of submergence were classified as “late response genes.” Next, we used Gene Ontology (GO)
enrichment analysis to elucidate the biological processes involved in regulating heterophylly (Fig. 4b and Supplementary Data 3). Among early response upregulated genes, those in the “shade
avoidance” category (GO-ID: 9641) were enriched, suggesting that light conditions are important in the early response to submergence. Significant numbers of both up- and down-regulated genes
were related to phytohormones, such as ethylene (GO-ID: 9723), gibberellin (GO-ID: 9739), and abscisic acid (ABA) (GO-ID: 9737). Some down-regulated genes were enriched in “response to
auxin stimulus” (GO-ID: 9641). The results are consistent with those of an earlier study18, which reported that phytohormones regulate heterophylly. Genes related to leaf morphology such as
leaf development (GO-ID: 48366) and leaf morphogenesis (GO-ID: 9965) were downregulated in response to submergence. Those involved in adaxial/abaxial axis specification (GO-ID: 9943) were
downregulated during the late response. Regulation of cell division and elongation is essential in altering leaf morphology. At the late stage, when the leaf morphology differed between the
submerged and terrestrial leaves, genes belonging to the cell cycle (GO-ID: 7049) and cell division (GO-ID: 51301) categories were enriched among the downregulated genes. The results
indicate that the expression of genes involved in leaf development is regulated immediately after submergence and that phytohormones are involved in the regulation. ETHYLENE INDUCES THE
SUBMERGED LEAF PHENOTYPE The results of the gene expression analyses suggested that ethylene, gibberellin, and abscisic acid are involved in the regulation of heterophylly. Our earlier
studies already showed that gibberellins are involved in the regulation of leaf morphology in _R. aquatica_3. Therefore, we examined the effect of ethylene and ABA on leaf shape in _R.
aquatica_. In numerous plant species, the accumulation of ethylene in plant tissues during submergence triggers the submergence response; for instance, ethylene participates in heterophylly
in some plant species5. Treatment of terrestrial _R. aquatica_ plants with 1-aminocyclopropane-1-carboxylic acid (ACC), an ethylene precursor, resulted in the formation of more deeply lobed
leaves with narrower leaf blades than that in the untreated terrestrial plants (Fig. 5a). Contrastingly, when the ethylene-response inhibitor AgNO3 was added under submerged conditions,
leaves with expanded leaf blades formed similar to the terrestrial leaves (Fig. 5b). When the ethylene concentration was increased, narrower leaf blades developed (Fig. 5c). The results
suggest that heterophylly in _R. aquatica_ is regulated by the concentration of the hormone ethylene. Furthermore, the submerged phenotype of leaves was suppressed by the inhibition of
ethylene response even under submerged conditions, suggesting that the ethylene response pathway mediates the regulation of heterophylly. ABA is a well-known hormone involved in the drought
response34 and is also known to be involved in the regulation of heterophylly in several amphibious plant species4,5,6,13. ABA treatment also inhibits _R. aquatica_ submergence response in
stomatal development33. We found that exogenous ABA treatment caused inhibition of leaf shape change in response to submergence (Supplementary Fig. 7). This result indicates that ABA is
involved in the regulation of heterophylly in _R. aquatica_, although long-term effects could not be observed because ABA treatment under submerged conditions strongly suppresses plant
growth. Next, we performed RNA-seq analysis of ACC-treated plants to identify the genes responsible for forming submerged type leaves. Because ACC treatment induced the formation of
submerged-type leaves, we extracted genes whose expression changed under both submerged and ACC-treated conditions (Fig. 5d, e and Supplementary Data 4): 143 and 82 genes were commonly
upregulated and downregulated, respectively. As expected, several common ethylene response genes were upregulated in both datasets. Contrastingly, submergence with AgNO3 showed an inversed
expression pattern as correlated to leaf morphology. One of the commonly regulated genes, _LONGIFOLIA_ (_LNG1_ and _LNG2_), was identified to participate in leaf morphogenesis in _A.
thaliana_ via activation-tag gene screening35: The dominant mutant of _LNG1_ (_lng1-1D_) formed elongated and narrow leaves with serrated margins; furthermore, _LNG1_ and _LNG2_ may function
redundantly and regulate the longitudinal elongation of cells. _LATE MERISTEM IDENTITY1_ (_LMI1)_ and _REDUCED COMPLEXITY_ (_RCO_) arose from the same ancestral gene via gene duplication
within a clade of Brassicaceae36. _RCO_ controls leaf complexity in _C. hirsuta_, wherein the wild type has compound leaves, even though the _rco_ mutant displays simple lobed leaves36.
_RCO_ is lost and _LMI1_ is retained in _A. thaliana_, resulting in simple leaves and the introduction of _C. hirsuta RCO_ into _A. thaliana_ resulted in the formation of serrations. This
_R. aquatica_ gene _RaChr03G09000_, extracted as an ortholog of _A. thaliana LMI1_, is an _RCO_ ortholog because it has a higher protein identity with _C. hirsuta RCO_ (84.3%) than with _C.
hirsuta LMI1_ (63.1%). Therefore, the upregulation of _R. aquatica RCO_ may cause the formation of compound leaves. _PHABULOSA_ (_PHB_), a member of the class III HD-ZIP gene family, directs
cells to adaxialize. Its dominant mutant, _phb-1d_, forms adaxialized radial leaves37. Loss of function of _PHB_ and other related class III HD-ZIP genes results in abaxialized radial
cotyledons38. The establishment of adaxial–abaxial polarity is required for leaf blade expansion, and loss of this polarity leads to leaf radialization. The involvement of adaxial–abaxial
polarity regulation in heterophylly was reported for _Ranunculus trichophyllus_6: submergence upregulated _KANADIs_ genes which regulates abaxial growth, resulting in the formation of
abaxialized radial leaves. In the present study, the expression of most adaxial genes, including _PHB_, were down-regulated under submergence and ACC-treated conditions (Fig. 5f). Regarding
abaxial genes, one of the _KAN1_ and _KAN3_ paralogs were significantly upregulated after 4 days of submergence. These results suggested that loss of polarity to abaxialization may be
involved in the formation of submerged-type leaves. Contrarily, other abaxial genes, _YABBYs_ (_YABs_) and _ARF3_ were downregulated in response to submergence. BLUE LIGHT INHIBITS THE
SUBMERGENCE SIGNAL In addition to genes related to leaf morphogenesis, genes in the GO categories “response to light stimulus” and “shade avoidance” were also affected by submergence (Fig.
4b). Submergence alters light quality through absorption and reflection in water and light quality affects various physiological responses in plants. Therefore, we investigated the effect of
light quality on leaf form under different light conditions. Blue light induced a pronounced response by elongating leaves along the anterior–posterior axis and preventing leaflet narrowing
in response to submergence (Fig. 6a). The Dissection Index (calculated from perimeter/square root of leaf area), as an indicator of leaf complexity, was subjected to statistical analysis
(Supplementary Fig. 8). No significant difference in leaf complexity was detected between terrestrial leaves under white and blue light conditions. Under white light condition, submerged
leaves showed high complexity with significant differences between terrestrial leaves. However, submergence under blue light caused an increase in complexity, and leaves with significant
lower complexity than that under white light condition were formed. Our transcriptome analysis comparing the effects of white and blue lights revealed that the expression profile of the
submerged leaves under blue light was negatively correlated with that under white light (Fig. 6b). This suggests that gene regulation normally induced during submergence under white light is
not induced by submergence under blue light. Particularly, the expression of ethylene response genes induced by submergence decreased under blue light (Supplementary Fig. 9). In addition,
the expression of genes regulating abaxial–adaxial polarity was not reduced under blue light. These results indicate that the submergence signal was inhibited under blue light conditions via
the ethylene response pathway. DISCUSSION Chromosome-level genome assembly and comparative genomic analysis reveal the genome structure of _R. aquatica_ and shed light on its origin and
evolution. We found that the genome of _R. aquatica_ arose by allotetraploidization through hybridization between two ancestral _Rorippa_ species (Fig. 3). Hybridization occurred no earlier
than 4.2 Mya when _R. aquatica_ subgenome A diverged from _R. islandica_. Thus, the genome of _R. aquatica_ arose by hybridization between two _Rorippa_ genomes with eight pairs of
chromosomes. WGD was followed by post-polyploid descending dysploidy (from _n_ = 16 to _n_ = 15) mediated by nested chromosome insertion (NCI), forming the fusion chromosome RaChr15. NCIs
(reduction from _n_ = 16 to _n_ = 15) also occurred in some populations of the tetraploid _Cardamine pratensis_39. Both NCI events in _Rorippa_ and _Cardamine_ involved chromosome AK8/6 as
the recipient chromosome, recombining with chromosome AK2 to form chromosome RaChr15 in _R. aquatica_, and chromosome AK5 in _C. pratensis_. An even more advanced post-polyploid descending
dysploidy was documented in the tetraploid _C. cordifolia_, where chromosome number was reduced from _n_ = 16 to _n_ = 12 due to the formation of five fusion chromosomes40. Contrastingly,
the closely related tetraploid genomes (2_n_ = 4x = 32) of horseradish (_Armoracia rusticana_) and watercress (_Nasturtium officinale_) contain structurally conserved parental subgenomes,
except for a 2.4-Mb long unequal translocation in watercress41. In Brassicaceae, polyploidization is not a rare event. Three allotetraploid _Brassica_ species arose from the hybridization of
two out of three diploid species42,43,44. In _Cardamine_, identification of polyploid origin were well proceeded with CCP and next-generation sequencing technology. These studies uncovered
potential parental species of polyploids and their evolutional process39,45. In _Rorippa_ genus, several polyploidizations in species- and intraspecific population-level is suggested based
on the variation of chromosome number46,47. Contrarily, their origin and evolutional process are not identified yet. The genome sequence of _R. aquatica_ provides a genome-wide reference for
future studies on _R. aquatica_, the entire genus _Rorippa_, and tribe cardaminoid (Cardamineae) genomes. Our transcriptome analysis of _R. aquatica_, based on whole-genome assembly data,
provides three key insights into heterophylly (Fig. 6c). First, the submergence signal is transmitted via ethylene, and ethylene signaling inhibits leaf blade expansion. We found that
ethylene signaling was induced by submergence and that exogenous ethylene resulted in narrower leaves even out of water. These responses were also observed in other amphibious plants4,6,13.
Ethylene signaling is a conserved pathway among angiosperms48, and ethylene is utilized as a submergence signal owing to its accumulation in response to submergence via low gaseous diffusion
in water. This function of ethylene is consistent with its apparent role in regulating heterophylly in response to submergence in various plant species. ABA is reported as a correlated
factor with ethylene response. The synthesis process and response pathway of ethylene and ABA regulate each other antagonistically49. In amphibious plants, up- and down-regulations of
ethylene and ABA in response to submergence and inhibition of submergence responses by exogenous ABA treatment were reported4,5,6,13. In _R. aquatica_, ABA responsive genes were highly
enriched in downregulated DEG at early response to submergence and exogenous ABA treatment inhibited submergence-responded heterophylly. Due to complex relationships between ethylene and
ABA, the function of ABA in submergence-related heterophylly have not been elucidated yet. Further analyses are essential to demonstrate whether ABA works as an inhibitor of ethylene or
plays an independent role in submergence responses. Gibberellins are also involved in regulating leaf form in _R. aquatica_, both under terrestrial and submerged conditions3. Gibberellin
treatment resulted in the emergence of simple leaves at low temperatures and submergence, conditions that normally result in dissected leaves, and inhibition of gibberellin signaling
resulted in dissected leaves even at high temperatures that normally induce simple leaves. While the effect of gibberellin content for morphology were identified, its molecular responses to
submergence were unknown. This study showed the change of genes related to the gibberellin stimulus in response to submergence. GO analysis indicated the enrichment of gibberellin responsive
genes in upregulated DEG as throughout and late responses and downregulated DEG as early response. The expression profile seems to increase gibberellin signaling in response to submergence
at least in the late response. Gibberellins showed different effects in other amphibious plants that do not show apparent heterophylly in response to temperature under terrestrial
conditions. In _Hygrophila difformis_ and _Callitriche palustris_, inhibition of gibberellin signaling suppresses the formation of the submerged-leaf phenotype, and gibberellin treatment
fails to induce this leaf phenotype under terrestrial conditions4,13. This suggests that leaf shape in _R. aquatica_ may be regulated by two parallel pathways: submergence-responsive
heterophylly mediated by ethylene and temperature-dependent heterophylly mediated by gibberellins. How the two pathways are regulated and interact is a topic for future work. Our second
important finding is that in _R. aquatica_, most adaxial polarity related genes were downregulated and some _KANs_ of abaxial genes were upregulated under submergence. These results
suggested that leaves narrowed down through abaxialization in response to submergence similar to that in _Ranunculus trichophyllus_6. However, no obvious morphological abaxialization, such
as radialization of vein in _R. aquatica_ submerged leaf, was observed in a previous study3, which is similar to that in _Hygrophila difformis_. Its submerged leaves having narrow lamina
kept normal polarity in vein and development of palisade tissue in adaxial side4. The establishment of adaxial–abaxial polarity eventually leads to the establishment of the middle domain,
which is located at the juxtaposition between the adaxial–abaxial domains and participates in the leaf lamina outgrowth50. The radialized narrow leaves shown in _A. thaliana_ polarity gene
mutants37 and submerged _Ranunculus trichophyllus_6 developed only main vein. Contrarily, highly dissected submerged leaves in _R. aquatica_ developed lateral veins. Temporal and spatial
regulation of leaf polarity may form dissected complex leaves in _R. aquatica_. A third important finding of our study is that blue light is involved in regulating heterophylly. At the gene
expression level, blue light blocked the upregulation of ethylene response genes during submergence. A similar effect of blue light was observed in submergence responses of stomatal
development in _R. aquatica_33. Submergence with white or red light promotes the expression of ethylene synthesis-related genes and inhibits stomatal development. Contrarily, these
submergence responses were inhibited under blue light. Although the mechanism by which blue light regulates heterophylly through ethylene is unclear, the relationship between blue light and
ethylene in the shade avoidance response has been studied. For example, in _A. thaliana_, low blue light induces stem elongation for shade avoidance, although not in ethylene-insensitive
mutants51. The molecular mechanisms underlying the response pathways to blue light and ethylene are unclear, even in _A. thaliana_. In plants, blue-light reception is mediated by the
cryptochromes (CRYs)52. In _Brassica napus_, overexpression of _CRY1_ leads to downregulation of _1-aminocyclopropane-1-carboxylate synthase 5_ and _8_ genes associated with ethylene
biosynthesis53. Changes in light quality and quantity do not seem to be the main factors of submergence signals, owing to only a small difference being caused by experimental submergence
treatment33. However, under natural conditions, the quality of light reaching submerged plants changes with water level and condition due to differences in the light-absorbance ratio.
Therefore, changes in light quality could provide coordinator signals about submerged conditions. Our results show that blue light plays an important role in regulating heterophylly in
response to submergence. Considering that the amphibious fern _Marsilea quadrifolia_ showed a similar response54, blue light signaling may also be central to heterophylly in various plants.
Paralogs related to ethylene response or adaxial–abaxial polarization showed similar expression patterns in response to environmental stimuli (Fig. 5f and Supplementary Fig. 8). This
suggests that a change in function or expression in the upstream regulatory gene causes the heterophylly upon submergence. The allotetraploid origin of _R. aquatica_ suggests several
possible mechanisms by which the species acquired traits such as amphibiousness and heterophylly in response to various signals. A most simple hypothesis is the inheritance from either
parent. However, this possibility is quite low because only _R. aquatica_ shows amphibiousness and remarkable heterophylly in _Rorippa_ genus. As another possibility, the hybridization of
two _Rorippa_ species at the first step of allotetraploidization may cause enhanced function like heterosis. The most likely hypothesis is the achievement of new functions caused by WGD.
Furthermore, the redundancy of duplicated genes often enables genes to acquire novel functions. Finally, accelerated accumulation of mutations could have led to specific traits. Future
comparative studies in the genus _Rorippa_ will determine the origin of _R. aquatica_ subgenomes and elucidate the evolution of adaptive mechanisms in a submerged condition. METHODS PLANT
MATERIALS _Rorippa aquatica_ plants (two accessions, N and S)18 were kept in a growth chamber at 30 °C under continuous light at 50 µmol photons m−2 s−1 supplied by a fluorescent lamp. For
each treatment, plants that regenerated from a leaf tip as described earlier19 were used. To induce inflorescences for comparative chromosome painting, the growth chamber temperature for _R.
aquatica_ accession S was changed to 20 °C. Young inflorescences were collected from plants and fixed in freshly prepared fixative (ethanol: acetic acid, 3:1) overnight, transferred to 70%
ethanol, and subsequently stored at −20 °C. For sequencings of genome, _R. aquatica_ accession N plants were grown in a growth chamber at 30 °C and used to extract genomic DNA. For
morphological and transcriptome analyses, _R. aquatica_ accession N plants were used. All plants used for these analyses were grown in a growth chamber at 25 °C. Plants used to examine the
change of morphology and gene expression after the transition to the submerged condition were grown in glass tanks with an approximate 8 cm water depth. To examine effects of ethylene on
heterophylly, the plants were treated with 100 µM 1-aminocyclopropane-1-carboxylic acid (ACC) or 1 µM AgNO3 by supplement of water containing chemical. For chemical treatment under submerged
conditions, the chemicals to be tested were diluted in the 200 mL sterile distilled water in the culture jar. For morphological analysis, plants were grown under each treatment for two
months until the leaves were mature. To examine the effect of ethylene amount, plants were treated with different concentrations of ACC (10, 100, and 1000 µM) under the terrestrial condition
described above. To examine effects of ABA on heterophylly, the plants were transfer to submerged condition containing 10 µM ABA and grown for 3 weeks. To examine the effects of blue light,
plants were grown under 20 µmol photons m−2 s−1 supplied by a blue LED. For RNA-seq analyses, plants grown under terrestrial condition with white light at 25 °C were transferred to each
experimental condition and grown at certain period (ACC: 1 day, AgNO3: 1 h, blue or white light: 1 h), and subjected to RNA extraction. CHROMOSOME PREPARATION Chromosome spreads from fixed
young flower buds containing immature anthers were prepared according to published protocols55,56. Chromosome preparations were treated with 100 µg/mL RNase in 2× sodium saline citrate (SSC,
20× SSC: 3 M sodium chloride, 300 mM trisodium citrate, pH 7.0) for 60 min, and with 0.1 mg/mL pepsin in 0.01 M HCl at 37 °C for 5 min, then post-fixed in 4% formaldehyde in distilled water
and dehydrated via an ethanol series (70%, 90%, and 100%, 2 min each). PAINTING PROBES For comparative chromosome painting (CCP), 674 chromosome-specific BAC clones of _Arabidopsis
thaliana_ (The Arabidopsis Information Resource, TAIR; http://www.arabidopsis.org) were used to establish contigs corresponding to the 22 genomic blocks (GBs) and eight chromosomes of the
Ancestral Crucifer Karyotype (ACK)24. To determine and characterize inversions of GBs on chromosome Ra15, BAC contigs corresponding to GBs D and E were split into smaller subcontigs and
differentially labeled and used in several consecutive experiments. All DNA probes were labeled with biotin-dUTP, digoxigenin-dUTP, or Cy3-dUTP by nick translation as described earlier57.
COMPARATIVE CHROMOSOME PAINTING DNA probes were pooled appropriately, ethanol precipitated, dried, and dissolved in 20 μL of 50% formamide and 10% dextran sulfate in 2× SSC. The dissolved
probe (20 μL) was pipetted onto a chromosome-containing slide and immediately denatured on a hot plate at 80 °C for 2 min. Hybridization was conducted in a moist chamber at 37 °C overnight.
Post-hybridization washing was performed in 20% formamide in 2× SSC at 42 °C. Hybridized probes were visualized either as the direct fluorescence of Cy3-dUTP or via fluorescently labeled
antibodies against biotin-dUTP and digoxigenin-dUTP57. Chromosomes were counterstained with 4′,6-diamidino-2-phenylindole (DAPI, 2 µg/mL) in Vectashield antifade (Vector Laboratories).
Fluorescence signals were analyzed and photographed using a Zeiss Axio Imager epifluorescence microscope with a CoolCube camera (MetaSystems, Altlussheim, Germany). Images were acquired
separately for all four fluorochromes using appropriate excitation and emission filters (AHF Analysentechnik, Tübingen, Germany). The four monochromatic images were pseudocolored, merged,
and cropped using Photoshop CS (Adobe Systems, Mountain View, CA) and ImageJ (National Institutes of Health, Bethesda, MA). ILLUMINA GENOME DNA SEQUENCING Genome-seq libraries were
constructed using whole- or nucleic-genome DNA. For extraction of nucleic DNA, the nuclear fraction was prepared from whole plants using the ‘Semi-pure Preparation of Nuclei Procedures’
protocol of the CelLytic PN Isolation/Extraction Kit (Sigma-Aldrich, St. Louis, MO). Next, genomic DNA was isolated from the nucleus or whole plant using a DNeasy Plant mini kit (Qiagen,
Hilden, Germany). Genome-seq libraries were prepared using the Nextera DNA Sample Prep Kit. Sequencing was performed using NextSeq 500, generating paired-end reads of 151 bp. PACBIO GENOME
DNA SEQUENCING DNA for PacBio library was prepared as follows: crude nuclei were obtained from regenerated plants (using ca. 1 cm lengths of leaf tip) using the ‘Crude Preparation of Nuclei
Procedures’ protocol of the CelLytic PN Isolation/Extraction Kit (Sigma-Aldrich). DNA extraction from crude nuclei was performed using two different methods. For the first run, the Dneasy
Plant mini kit was used. For the subsequent two runs, genomic DNA was extracted using phenol/chloroform/isoamyl alcohol extraction with CTAB buffer and purified using QIAGEN Genomic-tip
20/G. Long reads were generated using the PacBio RS II system. HI-C SEQ Hi-C Seq sample was prepared following a method reported in an earlier study58. HindIII was used for DNA digestion.
For the preparation of the sequencing library, the purified Hi-C sample (500 ng) was diluted to 500 µl with dH2O, and 500 µl of 2× binding buffer (BB) (10 mM Tris, 1 mM EDTA, 2 M NaCl) was
added. The diluted Hi-C samples were fragmented to a mean size of 300 bp by sonication using a Covaris M220 sonication system (Covaris, Woburn, MA, USA) in a milliTUBE 1 ml AFA Fibre
(Covaris). The parameters of the program were as follows: power mode, frequency sweeping; time, 20 min; duty cycle, 5%; intensity, 4; cycles per burst, 200; temperature (water bath), 6 °C.
Biotin-labeled Hi-C samples were then enriched using MyOne Streptavidin C1 magnetic beads (Veritas, Tokyo, Japan). For this, 60 µl of streptavidin beads were washed twice with 400 µl of
Tween Wash Buffer (TWB) (5 mM Tris, 0.5 mM EDTA, 1 M NaCl, 0.05% Tween-20). The recovery of streptavidin beads was performed by placing the tubes on a magnetic stand. Subsequently, the beads
were added to 1 ml of sheared Hi-C sample. After 15 min of incubation at room temperature under rotation, the supernatant was removed, and the beads binding biotinylated Hi-C fragments were
resuspended in 400 µl of 1× BB. Then, the beads were washed once in 60 µl RSB (Resuspension buffer) (Illumina, San Diego, CA, USA) and resuspended in 50 µl RSB. The enriched biotinylated
DNA fragments were subjected to library construction on beads using the KAPA HyperPrep Kit for Illumina (Roche, Basel, Switzerland) according to the manufacturer’s protocol, with 18 cycles
of PCR for library amplification. The amplified DNA fraction (50 µl) was corrected and purified using Agencourt AMPure XP (Beckman Coulter) following the standard protocol and finally
resuspended in 15 µl of RSB. The library was sequenced using a NextSeq 500 system, generating paired-end reads of 151 bp. GENOME SIZE ESTIMATION The genome size of _R. aquatica_ was
estimated by k-mer counting using jellyfish2 (http://www.genome.umd.edu/jellyfish.html). K-mers from Illumina read data were counted, and the k-mer distribution was plotted; the distribution
peaks from homozygous regions were picked manually, and genome size (in bases) was calculated as a total number of k-mers/peak of the k-mer distribution. GENOME ASSEMBLY AND ANNOTATION
Genome assembly was performed using MaSuRCA26 with both Illumina and PacBio reads. The assembled scaffolds were error-corrected using Pilon59. Scaffolding into chromosome-level sequences was
performed via the 3D de novo assembly (3D-DNA) pipeline60, using the assembled scaffolds and Hi-C Seq reads. The remaining gaps in chromosome-level sequences were filled by LR_Gapcloser61,
using PacBio reads that were error-corrected using ColorMap62. Assembled genome sequences were benchmarked using Benchmarking Universal Single-Copy Orthologs (BUSCO)63 with a land-plant
dataset (embryophyta_odb9). Repeat sequences in the genome were identified and masked using RepeatModeler and RepeatMasker (http://www.repeatmasker.org). Gene prediction was performed using
the PASA pipeline28. Three types of prediction were used: 1) ab initio prediction using AUGUSTUS64, GlimmerHMM65, and SNAP, with an _Arabidopsis_ training dataset; 2) Protein homology
detection using EXONERATE with _A. thaliana_ TAIR10 protein data; and 3) Alignment of assembled transcripts to the genome. Transcriptome data were obtained by de novo assembly using
Trinity66, with RNA-seq data (DRA006777) from an earlier study19. All the predicted gene structures were integrated into the final gene data using EvidenceModeler (EVM)67 and PASA. Gene
Ontology terms were assigned to each transcript using Blast2GO68 based on the results of a BLASTP homology search against the non-redundant protein sequence (Nr) database and InterProScan.
GENOME STRUCTURE Alignment of _R. aquatica_ chromosome sequences to the _C. hirsuta_ genome was performed using MUMMER69. Genome structure (distribution of genes, long terminal repeats, and
links between paralogous genes) was illustrated using CIRCOS70. COMPARATIVE GENOMICS ANALYSIS We performed whole-genome level phylogenetic analysis using _R. aquatica_ genomic information
and genome-level data of several plant species. The protein dataset of 22 plant species were obtained from the Phytozome database (https://phytozome-next.jgi.doe.gov/). The datasets of _C.
hirsuta_ and _Barbarea vulgaris_ were prepared using data from the genome database of each species (http://chi.mpipz.mpg.de/71 and https://www.ebi.ac.uk/ena/browser/view/LXTM0100000072,
respectively). We prepared draft _Rorippa islandica_ genomic data via genome assembly using Velvet73 with genome-seq read data (SRR1801303) from the Sequence Read Archive, setting k-mer to
151. Using an Arabidopsis training dataset, gene prediction was performed using AUGUSTUS74. Protein sequences of a single representative longest transcript variant for each gene were
extracted using an inhouse Perl script. Using OrthoFinder75, each protein sequence was clustered into an orthogroup based on similarity, and the phylogenetic analysis of each orthogroup was
integrated as a species tree. The synonymous substitution rate (Ks) was calculated to estimate evolutionary event ages. Using MACSE76, we performed multiple-alignment of the coding DNA
sequences in each orthogroups in which single-copy conserved genes in seven related Brassicaceae species (_Eutrema salsugineum_, _Arabidopsis thaliana_, _Arabidopsis lyrata_, _Barbarea
vulgaris_, _Cardamine hirsuta_, _Capsella rubella_, and _Rorippa islandica_) and duplicated genes in _R. aquatica_ were classified, and then we calculated Ks using yn00 in the PAML package
(http://abacus.gene.ucl.ac.uk/software/paml.html). The age of each event was estimated as T (in years) = Ks of peak/(2 * _μ_), where _μ_, which is the synonymous divergence rate per site per
year, equals 6.51648E−09 in Brassicaceae32. TRANSCRIPTOME ANALYSIS Total RNA was isolated from shoot apexes containing young leaves using a RNeasy Plant Mini kit (QIAGEN, Hilden, Germany).
RNA-seq libraries were prepared using the Illumina TruSeq Stranded RNA LT kit (Illumina, CA, USA), according to the manufacturer’s instructions. Libraries were sequenced on the NextSeq500
sequencing platform (Illumina, CA, USA), and 76 bp single-end reads were obtained. The reads were mapped to the genome sequences of _R. aquatica_ using Tophat2. Count data were subjected to
a trimmed mean of M-value normalization in the edgeR77. Transcript expression and DEGs were defined using the edgeR GLM approach with cut off threshold, false discovery rate (FDR) < 0.01,
and |log2FC| > 1. Summary of RNA-seq read data and mapping are shown in Supplementary Data 5. STATISTICAL ANALYSIS OF LEAF COMPLEXITY To assess complexity of leaf form, Dissection Index
was calculated from morphological image using the following formula (perimeter / square root of leaf area). Perimeter and area of leaves were measured from photographs using ImageJ
software78. Statistical analysis was carried out using Tukey-Kramer test. STATISTICS AND REPRODUCIBILITY The information of statistical tests used in the study are provided in the respective
methods sections and Supplementary Data. The RNA-seq was performed in three independent biological replicates and statistically analyzed using the edgeR GLM approach. For leaf complexity,
8–21 leaves from each experimental condition were measured and mean dissection indexes were statistically analyzed using Tukey-Kramer test. REPORTING SUMMARY Further information on research
design is available in the Nature Portfolio Reporting Summary linked to this article. DATA AVAILABILITY The assembled _R. aquatica_ genome sequences and their annotations were deposited in
Figshare (10.6084/m9.figshare.19207362). The draft assembly data of _R. islandica_ were deposited in Figshare (10.6084/m9.figshare.24849201). Genome-seq read data and Hi-C seq read data are
available in the DDBJ Sequenced Read Archive (DRA) under the accession numbers DRA010675 and DRA013596, respectively. Transcriptome read data are also available in DRA under DRA014113,
DRA014114, DRA014164, and DRA014165. The numerical source data behind the graphs in Figs. 3a and 5f can be found in Supplementary Data 6. All other data are available from the corresponding
author on reasonable request. REFERENCES * Li, G., Hu, S., Hou, H. & Kimura, S. Heterophylly: phenotypic plasticity of leaf shape in aquatic and amphibious plants. _Plants Basel Switz._
8, E420 (2019). Google Scholar * Wells, C. L. & Pigliucci, M. Adaptive phenotypic plasticity: the case of heterophylly in aquatic plants. _Perspect. Plant Ecol. Evol. Syst._ 3, 1–18
(2000). Article Google Scholar * Nakayama, H. et al. Regulation of the KNOX-GA gene module induces heterophyllic alteration in North American lake cress. _Plant Cell_ 26, 4733–4748 (2014).
Article CAS PubMed PubMed Central Google Scholar * Li, G. et al. Water-Wisteria as an ideal plant to study heterophylly in higher aquatic plants. _Plant Cell Rep._ 36, 1225–1236
(2017). Article CAS PubMed Google Scholar * Kuwabara, A., Ikegami, K., Koshiba, T. & Nagata, T. Effects of ethylene and abscisic acid upon heterophylly in _Ludwigia arcuata_
(Onagraceae). _Planta_ 217, 880–887 (2003). Article CAS PubMed Google Scholar * Kim, J. et al. A molecular basis behind heterophylly in an amphibious plant, _Ranunculus trichophyllus_.
_PLoS Genet._ 14, e1007208 (2018). Article PubMed PubMed Central Google Scholar * Doll, Y., Koga, H. & Tsukaya, H. The diversity of stomatal development regulation in _Callitriche_
is related to the intrageneric diversity in lifestyles. _Proc. Natl Acad. Sci. USA_ 118, e2026351118 (2021). Article CAS PubMed PubMed Central Google Scholar * van Veen, H. &
Sasidharan, R. Shape shifting by amphibious plants in dynamic hydrological niches. _N. Phytol._ 229, 79–84 (2021). Article Google Scholar * Nakayama, H., Nakayama, N., Nakamasu, A., Sinha,
N. & Kimura, S. Toward elucidating the mechanisms that regulate heterophylly. _Plant Morphol._ 24, 57–63 (2012). Article Google Scholar * Nakayama, H., Sinha, N. R. & Kimura, S.
How do plants and phytohormones accomplish heterophylly, leaf phenotypic plasticity, in response to environmental cues. _Front. Plant Sci._ 8, 1717 (2017). Article PubMed PubMed Central
Google Scholar * Li, G. et al. Mechanisms of the morphological plasticity induced by phytohormones and the environment in plants. _Int. J. Mol. Sci._ 22, E765 (2021). Article Google
Scholar * Li, G. et al. Establishment of an Agrobacterium mediated transformation protocol for the detection of cytokinin in the heterophyllous plant _Hygrophila difformis_ (Acanthaceae).
_Plant Cell Rep._ 39, 737–750 (2020). Article CAS PubMed Google Scholar * Koga, H., Kojima, M., Takebayashi, Y., Sakakibara, H. & Tsukaya, H. Identification of the unique molecular
framework of heterophylly in the amphibious plant _Callitriche palustris_ L. _Plant Cell_ 33, 3272–3292 (2021). Article PubMed PubMed Central Google Scholar * La Rue, C. Regeneration in
Radicula aquatica. _Pap. Mich. Acad. Sci. Arts Lett._ 28, 51–61 (1943). Google Scholar * Nakayama, H., Fukushima, K., Fukuda, T., Yokoyama, J. & Kimura, S. Molecular phylogeny
determined using chloroplast DNA inferred a new phylogenetic relationship of _Rorippa aquatica_ (Eaton) EJ Palmer & Steyermark (Brassicaceae)—Lake Cress. _Am. J. Plant Sci._ 05, 48–54
(2014). Article CAS Google Scholar * Warwick, S. I., Francis, A. & Al-Shehbaz, I. A. Brassicaceae: species checklist and database on CD-Rom. _Plant Syst. Evol._ 259, 249–258 (2006).
Article Google Scholar * Flora of North America Editorial Committee. _Flora of North America: Volume 7: Magnoliophyta: Dilleniidae, Part 2_ (ed. Flora of North America Editorial Committee)
493–506 (Oxford University Press Inc, 2010). * Nakayama, H. et al. Comparative transcriptomics with self-organizing map reveals cryptic photosynthetic differences between two accessions of
North American Lake cress. _Sci. Rep._ 8, 3302 (2018). Article PubMed PubMed Central Google Scholar * Amano, R. et al. Molecular basis for natural vegetative propagation via regeneration
in North American Lake Cress, _Rorippa aquatica_ (Brassicaceae). _Plant Cell Physiol._ 61, 353–369 (2020). Article CAS PubMed Google Scholar * Les, D. H. Molecular systematics and
taxonomy of lake cress (_Neobeckia aquatica_; Brassicaceae), an imperiled aquatic mustard. _Aquat. Bot._ 49, 149–165 (1994). Article CAS Google Scholar * Hay, A. S. et al. _Cardamine
hirsuta_: a versatile genetic system for comparative studies. _Plant J. Cell Mol. Biol._ 78, 1–15 (2014). Article CAS Google Scholar * Bar, M. & Ori, N. Compound leaf development in
model plant species. _Curr. Opin. Plant Biol._ 23, 61–69 (2015). Article PubMed Google Scholar * Schranz, M. E., Lysak, M. A. & Mitchell-Olds, T. The ABC’s of comparative genomics in
the Brassicaceae: building blocks of crucifer genomes. _Trends Plant Sci._ 11, 535–542 (2006). Article CAS PubMed Google Scholar * Lysak, M. A., Mandáková, T. & Schranz, M. E.
Comparative paleogenomics of crucifers: ancestral genomic blocks revisited. _Curr. Opin. Plant Biol._ 30, 108–115 (2016). Article PubMed Google Scholar * Mandáková, T. et al. The story of
promiscuous crucifers: origin and genome evolution of an invasive species, _Cardamine occulta_ (Brassicaceae), and its relatives. _Ann. Bot._ 124, 209–220 (2019). Article PubMed PubMed
Central Google Scholar * Zimin, A. V. et al. Hybrid assembly of the large and highly repetitive genome of _Aegilops tauschii_, a progenitor of bread wheat, with the MaSuRCA mega-reads
algorithm. _Genome Res._ 27, 787–792 (2017). Article CAS PubMed PubMed Central Google Scholar * Haas, B. J. et al. Improving the Arabidopsis genome annotation using maximal transcript
alignment assemblies. _Nucleic Acids Res._ 31, 5654–5666 (2003). Article CAS PubMed PubMed Central Google Scholar * Haas, B. J. et al. Automated eukaryotic gene structure annotation
using EVidenceModeler and the Program to Assemble Spliced Alignments. _Genome Biol._ 9, R7 (2008). Article PubMed PubMed Central Google Scholar * Jeelani, S. M., Rani, S., Kumar, S.,
Kumari, S. & Gupta, R. C. Cytological studies of Brassicaceae burn. (Cruciferae juss.) from Western Himalayas. _Tsitol. Genet._ 47, 26–36 (2013). CAS PubMed Google Scholar * Huang,
C.-H. et al. Resolution of Brassicaceae phylogeny using nuclear genes uncovers nested radiations and supports convergent morphological evolution. _Mol. Biol. Evol._ 33, 394–412 (2016).
Article CAS PubMed Google Scholar * Cai, L. & Ma, H. Using nuclear genes to reconstruct angiosperm phylogeny at the species level: a case study with Brassicaceae species. _J. Syst.
Evol._ 54, 438–452 (2016). Article Google Scholar * De La Torre, A. R., Li, Z., Van de Peer, Y. & Ingvarsson, P. K. Contrasting rates of molecular evolution and patterns of selection
among gymnosperms and flowering plants. _Mol. Biol. Evol._ 34, 1363–1377 (2017). Article PubMed Google Scholar * Ikematsu, S. et al. Rewiring of hormones and light response pathways
underlies the inhibition of stomatal development in an amphibious plant _Rorippa aquatica_ underwater. _Curr. Biol._ 33, 543–556.e4 (2023). Article CAS PubMed Google Scholar * Kuromori,
T., Fujita, M., Takahashi, F., Yamaguchi-Shinozaki, K. & Shinozaki, K. Inter-tissue and inter-organ signaling in drought stress response and phenotyping of drought tolerance. _Plant J.
Cell Mol. Biol._ 109, 342–358 (2022). Article CAS Google Scholar * Lee, Y. K. et al. _LONGIFOLIA1_ and _LONGIFOLIA2_, two homologous genes, regulate longitudinal cell elongation in
_Arabidopsis_. _Development_ 133, 4305–4314 (2006). Article CAS PubMed Google Scholar * Vlad, D. et al. Leaf shape evolution through duplication, regulatory diversification, and loss of
a homeobox gene. _Science_ 343, 780–783 (2014). Article CAS PubMed Google Scholar * Eshed, Y., Izhaki, A., Baum, S. F., Floyd, S. K. & Bowman, J. L. Asymmetric leaf development and
blade expansion in _Arabidopsis_ are mediated by KANADI and YABBY activities. _Development_ 131, 2997–3006 (2004). Article CAS PubMed Google Scholar * Emery, J. F. et al. Radial
patterning of Arabidopsis shoots by class III HD-ZIP and KANADI genes. _Curr. Biol._ 13, 1768–1774 (2003). Article CAS PubMed Google Scholar * Mandáková, T. et al. The more the merrier:
recent hybridization and polyploidy in _Cardamine_. _Plant Cell_ 25, 3280–3295 (2013). Article PubMed PubMed Central Google Scholar * Mandáková, T., Gloss, A. D., Whiteman, N. K. &
Lysak, M. A. How diploidization turned a tetraploid into a pseudotriploid. _Am. J. Bot._ 103, 1187–1196 (2016). Article PubMed Google Scholar * Mandáková, T. & Lysak, M. A. Healthy
roots and leaves: comparative genome structure of horseradish and watercress. _Plant Physiol._ 179, 66–73 (2019). Article PubMed Google Scholar * Nagaharu, U. & Nagaharu, N. Genome
analysis in Brassica with special reference to the experimental formation of B. napus and peculiar mode of fertilization. _Jpn J. Bot._ 7, 389–452 (1935). Google Scholar * Chalhoub, B. et
al. Early allopolyploid evolution in the post-Neolithic Brassica napus oilseed genome. _Science_ 345, 950–953 (2014). Plant genetics. Article CAS PubMed Google Scholar * Yang, J. et al.
The genome sequence of allopolyploid _Brassica juncea_ and analysis of differential homoeolog gene expression influencing selection. _Nat. Genet._ 48, 1225–1232 (2016). Article CAS PubMed
Google Scholar * Šlenker, M. et al. Allele sorting as a novel approach to resolving the origin of allotetraploids using Hyb-Seq data: a case study of the Balkan mountain endemic Cardamine
barbaraeoides. _Front. Plant Sci._ 12, 659275 (2021). Article PubMed PubMed Central Google Scholar * Javůrková-Kratochvílo, T. P. Chromosome study of the genus _Rorippa_ Scop. em.
Reichenb. in Czechoslovakia. _Preslia_ 44, 140–156 (1972). * Howard, H. W. Chromosome numbers of British species of the genus _Rorippa_ Scop (part of the Genus _Nasturtium_ R.Br.). _Nature_
159, 66–66 (1947). Article Google Scholar * Binder, B. M. Ethylene signaling in plants. _J. Biol. Chem._ 295, 7710–7725 (2020). Article CAS PubMed PubMed Central Google Scholar *
Cheng, W.-H., Chiang, M.-H., Hwang, S.-G. & Lin, P.-C. Antagonism between abscisic acid and ethylene in Arabidopsis acts in parallel with the reciprocal regulation of their metabolism
and signaling pathways. _Plant Mol. Biol._ 71, 61–80 (2009). Article CAS PubMed PubMed Central Google Scholar * Nakata, M. et al. Roles of the middle domain-specific _WUSCHEL-RELATED
HOMEOBOX_ genes in early development of leaves in _Arabidopsis_. _Plant Cell_ 24, 519–535 (2012). Article CAS PubMed PubMed Central Google Scholar * Pierik, R., Whitelam, G. C.,
Voesenek, L. A. C. J., de Kroon, H. & Visser, E. J. W. Canopy studies on ethylene-insensitive tobacco identify ethylene as a novel element in blue light and plant-plant signalling.
_Plant J. Cell Mol. Biol._ 38, 310–319 (2004). Article CAS Google Scholar * Lin, C. Plant blue-light receptors. _Trends Plant Sci._ 5, 337–342 (2000). Article CAS PubMed Google Scholar
* Sharma, P., Chatterjee, M., Burman, N. & Khurana, J. P. Cryptochrome 1 regulates growth and development in _Brassica_ through alteration in the expression of genes involved in light,
phytohormone and stress signalling. _Plant Cell Environ._ 37, 961–977 (2014). Article CAS PubMed Google Scholar * Lin, B. L. & Yang, W. J. Blue light and abscisic acid independently
induce heterophyllous switch in _Marsilea quadrifolia_. _Plant Physiol._ 119, 429–434 (1999). Article CAS PubMed PubMed Central Google Scholar * Lysak, M. A. & Mandáková, T.
Analysis of plant meiotic chromosomes by chromosome painting. _Methods Mol. Biol. Clifton NJ_ 990, 13–24 (2013). Article CAS Google Scholar * Mandáková, T. & Lysak, M. A. Chromosome
preparation for cytogenetic analyses in _Arabidopsis_. _Curr. Protoc. Plant Biol._ 1, 43–51 (2016). Article PubMed Google Scholar * Mandáková, T. & Lysak, M. A. Painting of
Arabidopsis chromosomes with chromosome-specific BAC clones. _Curr. Protoc. Plant Biol._ 1, 359–371 (2016). Article PubMed Google Scholar * Grob, S. & Grossniklaus, U. Chromatin
conformation capture-based analysis of nuclear architecture. _Methods Mol. Biol. Clifton NJ_ 1456, 15–32 (2017). Article CAS Google Scholar * Walker, B. J. et al. Pilon: an integrated
tool for comprehensive microbial variant detection and genome assembly improvement. _PLoS ONE_ 9, e112963 (2014). Article PubMed PubMed Central Google Scholar * Dudchenko, O. et al. De
novo assembly of the _Aedes aegypti_ genome using Hi-C yields chromosome-length scaffolds. _Science_ 356, 92–95 (2017). Article CAS PubMed PubMed Central Google Scholar * Xu, G.-C. et
al. LR_Gapcloser: a tiling path-based gap closer that uses long reads to complete genome assembly. _GigaScience_ 8, giy157 (2019). Article PubMed Google Scholar * Haghshenas, E., Hach,
F., Sahinalp, S. C. & Chauve, C. CoLoRMap: correcting long reads by mapping short reads. _Bioinformatics_ 32, i545–i551 (2016). Article CAS PubMed Google Scholar * Simão, F. A.,
Waterhouse, R. M., Ioannidis, P., Kriventseva, E. V. & Zdobnov, E. M. BUSCO: assessing genome assembly and annotation completeness with single-copy orthologs. _Bioinformatics_ 31,
3210–3212 (2015). Article PubMed Google Scholar * Stanke, M. & Waack, S. Gene prediction with a hidden Markov model and a new intron submodel. _Bioinformatics_ 19, ii215–225 (2003).
Article PubMed Google Scholar * Salzberg, S. L., Pertea, M., Delcher, A. L., Gardner, M. J. & Tettelin, H. Interpolated Markov models for eukaryotic gene finding. _Genomics_ 59, 24–31
(1999). Article CAS PubMed Google Scholar * Grabherr, M. G. et al. Full-length transcriptome assembly from RNA-Seq data without a reference genome. _Nat. Biotechnol._ 29, 644–652
(2011). Article CAS PubMed PubMed Central Google Scholar * Allen, J. E., Pertea, M. & Salzberg, S. L. Computational gene prediction using multiple sources of evidence. _Genome Res._
14, 142–148 (2004). Article CAS PubMed PubMed Central Google Scholar * Conesa, A. & Götz, S. Blast2GO: a comprehensive suite for functional analysis in plant genomics. _Int. J.
Plant Genomics_ 2008, 619832 (2008). Article PubMed Google Scholar * Kurtz, S. et al. Versatile and open software for comparing large genomes. _Genome Biol._ 5, R12 (2004). Article
PubMed PubMed Central Google Scholar * Krzywinski, M. I. et al. Circos: an information aesthetic for comparative genomics. _Genome Res_. https://doi.org/10.1101/gr.092759.109 (2009). *
Gan, X. et al. The _Cardamine hirsuta_ genome offers insight into the evolution of morphological diversity. _Nat. Plants_ 2, 16167 (2016). Article CAS PubMed PubMed Central Google
Scholar * Byrne, S. L. et al. The genome sequence of _Barbarea vulgaris_ facilitates the study of ecological biochemistry. _Sci. Rep._ 7, 40728 (2017). Article CAS PubMed PubMed Central
Google Scholar * Zerbino, D. R. & Birney, E. Velvet: algorithms for de novo short read assembly using de Bruijn graphs. _Genome Res._ 18, 821–829 (2008). Article CAS PubMed PubMed
Central Google Scholar * Stanke, M., Schöffmann, O., Morgenstern, B. & Waack, S. Gene prediction in eukaryotes with a generalized hidden Markov model that uses hints from external
sources. _BMC Bioinforma._ 7, 62 (2006). Article Google Scholar * Emms, D. M. & Kelly, S. OrthoFinder: solving fundamental biases in whole genome comparisons dramatically improves
orthogroup inference accuracy. _Genome Biol._ 16, 157 (2015). Article PubMed PubMed Central Google Scholar * Ranwez, V., Harispe, S., Delsuc, F. & Douzery, E. J. P. MACSE: multiple
Alignment of Coding SEquences accounting for frameshifts and stop codons. _PLoS ONE_ 6, e22594 (2011). Article CAS PubMed PubMed Central Google Scholar * Robinson, M. D., McCarthy, D.
J. & Smyth, G. K. edgeR: a Bioconductor package for differential expression analysis of digital gene expression data. _Bioinformatics_ 26, 139–140 (2010). Article CAS PubMed Google
Scholar * Schneider, C. A., Rasband, W. S. & Eliceiri, K. W. NIH Image to ImageJ: 25 years of image analysis. _Nat. Methods_ 9, 671–675 (2012). Article CAS PubMed PubMed Central
Google Scholar Download references ACKNOWLEDGEMENTS We thank Dr. Neelima Sinha, Dr. Naomi Nakayama, and Dr. Dhanya Radhakrishnan for valuable discussions. This work was financially
supported by the JSPS KAKENHI grants 21H02513 and MEXT-Supported Program for the Strategic Research Foundation at Private Universities grant S1511023 to S.K. and JSPS KAKENHI grants 20H05911
and 22H00415 to S.M. This work was also supported by the National Key Research and Development Program of China (2017YFE0128800), the National Natural Science Foundation of China (31870384,
32101254), and the International Partnership Program of the Chinese Academy of Sciences (152342KYSB20200021) to H.H. and G.L., and by the project TowArds Next GENeration Crops (no. 17
CZ.02.01.01/00/22_008/0004581) of the ERDF Programme Johannes Amos Comenius to M.A.L and T.M. AUTHOR INFORMATION Author notes * Takuya Sakamoto Present address: Faculty of Science, Kanagawa
University, 3-27-1 Rokkakubashi, Kanagawa-ku, Yokohama, Kanagawa, Japan AUTHORS AND AFFILIATIONS * Faculty of Life Sciences, Kyoto Sangyo University, Kamigamo-Motoyama, Kita-ku, Kyoto, Japan
Tomoaki Sakamoto, Shuka Ikematsu, Hokuto Nakayama & Seisuke Kimura * Center for Plant Sciences, Kyoto Sangyo University, Kamigamo-Motoyama, Kita-ku, Kyoto, Japan Tomoaki Sakamoto, Shuka
Ikematsu & Seisuke Kimura * Graduate School of Science, Department of Biological Sciences, The University of Tokyo, Science Build. #2, 7-3-1 Hongo, Bunkyo-ku, Tokyo, Japan Hokuto
Nakayama * Department of Plant Biology, University of California Davis, One Shields Avenue, Davis, CA, USA Hokuto Nakayama * CEITEC – Central European Institute of Technology, Masaryk
University, CZ-625 00, Brno, Czech Republic Terezie Mandáková & Martin A. Lysak * Department of Horticulture, Faculty of Agriculture, University of Maragheh, Maragheh, Iran Gholamreza
Gohari * Department of Applied Biological Science, Faculty of Science and Technology, Tokyo University of Science, 2641 Yamazaki, Noda, Chiba, Japan Takuya Sakamoto * The Key Laboratory of
Aquatic Biodiversity and Conservation of Chinese Academy of Sciences, Institute of Hydrobiology, Chinese Academy of Sciences, Wuhan, Hubei, China Gaojie Li & Hongwei Hou * Department of
Integrated Biosciences, Graduate School of Frontier Science, The University of Tokyo, Chiba, Japan Sachihiro Matsunaga Authors * Tomoaki Sakamoto View author publications You can also search
for this author inPubMed Google Scholar * Shuka Ikematsu View author publications You can also search for this author inPubMed Google Scholar * Hokuto Nakayama View author publications You
can also search for this author inPubMed Google Scholar * Terezie Mandáková View author publications You can also search for this author inPubMed Google Scholar * Gholamreza Gohari View
author publications You can also search for this author inPubMed Google Scholar * Takuya Sakamoto View author publications You can also search for this author inPubMed Google Scholar *
Gaojie Li View author publications You can also search for this author inPubMed Google Scholar * Hongwei Hou View author publications You can also search for this author inPubMed Google
Scholar * Sachihiro Matsunaga View author publications You can also search for this author inPubMed Google Scholar * Martin A. Lysak View author publications You can also search for this
author inPubMed Google Scholar * Seisuke Kimura View author publications You can also search for this author inPubMed Google Scholar CONTRIBUTIONS To.S., S.I., H.N., T.M., and Ta.S.
performed the experiments; To.S. and S.K. carried out the bioinformatic analyses; To.S., H.N., T.M., G.G., Ta.S., G.L., M.A.L., and K.S. wrote the article with input from all authors; H.H.,
S.M., M.A.L., and K.S. supervised; all authors discussed and commented on the article. CORRESPONDING AUTHOR Correspondence to Seisuke Kimura. ETHICS DECLARATIONS COMPETING INTERESTS The
authors declare no competing interests. PEER REVIEW PEER REVIEW INFORMATION _Communications Biology_ thanks the anonymous reviewers for their contribution to the peer review of this work.
Primary Handling Editors: Diane Saunders and David Favero. A peer review file is available. ADDITIONAL INFORMATION PUBLISHER’S NOTE Springer Nature remains neutral with regard to
jurisdictional claims in published maps and institutional affiliations. SUPPLEMENTARY INFORMATION PEER REVIEW FILE SUPPLEMENTARY INFORMATION DESCRIPTION OF ADDITIONAL SUPPLEMENTARY FILES
SUPPLEMENTARY DATA 1. ORTHOLOG CLUSTERING IN RORIPPA AND BRASSICACEAE SUPPLEMENTARY DATA 2. RNA-SEQ EXPRESSION PROFILE DATA SUPPLEMENTARY DATA 3. GO ENRICHMENT ANALYSIS FOR DEG AT
SUBMERGENCE SUPPLEMENTARY DATA 4. COMMON DEGS INDUCING SUBMERGED-TYPE LEAF SUPPLEMENTARY DATA 5. INFORMATION OF RNASEQ READ AND MAPPING STATISTICS SUPPLEMENTARY DATA 6. NUMERICAL SOURCE DATA
FOR GRAPHS REPORTING SUMMARY RIGHTS AND PERMISSIONS OPEN ACCESS This article is licensed under a Creative Commons Attribution 4.0 International License, which permits use, sharing,
adaptation, distribution and reproduction in any medium or format, as long as you give appropriate credit to the original author(s) and the source, provide a link to the Creative Commons
licence, and indicate if changes were made. The images or other third party material in this article are included in the article’s Creative Commons licence, unless indicated otherwise in a
credit line to the material. If material is not included in the article’s Creative Commons licence and your intended use is not permitted by statutory regulation or exceeds the permitted
use, you will need to obtain permission directly from the copyright holder. To view a copy of this licence, visit http://creativecommons.org/licenses/by/4.0/. Reprints and permissions ABOUT
THIS ARTICLE CITE THIS ARTICLE Sakamoto, T., Ikematsu, S., Nakayama, H. _et al._ A chromosome-level genome assembly for the amphibious plant _Rorippa aquatica_ reveals its allotetraploid
origin and mechanisms of heterophylly upon submergence. _Commun Biol_ 7, 431 (2024). https://doi.org/10.1038/s42003-024-06088-7 Download citation * Received: 07 March 2023 * Accepted: 21
March 2024 * Published: 18 April 2024 * DOI: https://doi.org/10.1038/s42003-024-06088-7 SHARE THIS ARTICLE Anyone you share the following link with will be able to read this content: Get
shareable link Sorry, a shareable link is not currently available for this article. Copy to clipboard Provided by the Springer Nature SharedIt content-sharing initiative