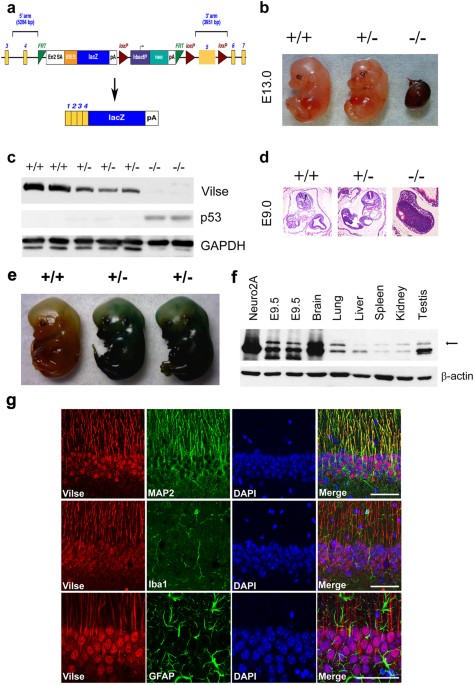
Important roles of vilse in dendritic architecture and synaptic plasticity
- Select a language for the TTS:
- UK English Female
- UK English Male
- US English Female
- US English Male
- Australian Female
- Australian Male
- Language selected: (auto detect) - EN
Play all audios:

ABSTRACT Vilse/Arhgap39 is a Rho GTPase activating protein (RhoGAP) and utilizes its WW domain to regulate Rac/Cdc42-dependent morphogenesis in Drosophila and murine hippocampal neurons.
However, the function of Vilse in mammalian dendrite architecture and synaptic plasticity remained unclear. In the present study, we aimed to explore the possible role of Vilse in dendritic
structure and synaptic function in the brain. Homozygous knockout of _Vilse_ resulted in premature embryonic lethality in mice. Changes in dendritic complexity and spine density were noticed
in hippocampal neurons of Camk2a-Cre mediated forebrain-specific Vilse knockout (VilseΔ/Δ) mice. VilseΔ/Δ mice displayed impaired spatial memory in water maze and Y-maze tests. Electrical
stimulation in hippocampal CA1 region revealed that the synaptic transmission and plasticity were defected in VilseΔ/Δ mice. Collectively, our results demonstrate that Vilse is essential for
embryonic development and required for spatial memory. SIMILAR CONTENT BEING VIEWED BY OTHERS MAF1 REGULATES DENDRITIC MORPHOGENESIS AND INFLUENCES LEARNING AND MEMORY Article Open access
30 July 2020 THE CYTOPLASMIC LOCALIZATION OF ADNP THROUGH 14-3-3 PROMOTES SEX-DEPENDENT NEURONAL MORPHOGENESIS, CORTICAL CONNECTIVITY, AND CALCIUM SIGNALING Article 11 January 2023 LOSS OF
RYANODINE RECEPTOR 2 IMPAIRS NEURONAL ACTIVITY-DEPENDENT REMODELING OF DENDRITIC SPINES AND TRIGGERS COMPENSATORY NEURONAL HYPEREXCITABILITY Article Open access 08 July 2020 INTRODUCTION Rho
GTPases are involved in cell dynamics, cell growth, intracellular membrane trafficking, and apoptosis1. Among numerous identified Rho GTPases, the most known members are RhoA (Ras
homologous member A), Rac1 (Ras-related C3 botulinum toxin substrate 1) and Cdc42 (cell division cycle 42). Rho GTPases switch between an active GTP-bound form and an inactive GDP-bound
state. Two classes of proteins are responsible for the activation and inactivation of Rho GTPases. Guanine nucleotide exchange factors (GEFs) control GTPase activation by catalyzing the
exchange of GDP for GTP2. By contrast, GTPase activating proteins (GAPs) increase the intrinsic GTPase activity of Rho GTPases to facilitate the hydrolysis of Rho-GTP, thereby switching them
to inactive Rho GTPases3. Most excitatory synapses in the mammalian brain locate at dendritic spines and the formation and dynamics of dendritic spines are mainly determined by the actin
cytoskeleton4. Rho GTPases play important roles in the morphogenesis of dendritic spines and synaptic plasticity by modulating the actin organization5,6,7,8. RhoA is essential for stress
fiber formation, whereas Rac and Cdc42 regulate the formation of lamellipodia and filopodia, respectively9. In particular, Cdc42 and Rac1 promote the formation and maintenance of dendritic
spines, while as RhoA negatively regulates spinogenesis10,11. Morphological analysis revealed a significant decrease of spine density in the CA1 pyramidal neurons of Camk2a-cre medicated
Cdc42 knockout mice12. Similarly, elimination of Rac1 in excitatory neurons in the forebrain _in vivo_ affects spine structure and impairs synaptic plasticity in the hippocampus with defects
in spatial learning13. In contrast, RhoA inhibition increases spine density and length and constitutively active RhoA mutant resulted in the opposite effects of RhoA ablation on dendritic
spines14. Vilse/Arhgap39 is a multidomain protein containing WW domain (a.a 65–95), MyTH4 (myosin tail homolog 4) domain (a.a. 760–875), and RhoGAP domain (a.a. 940–1085) for inactivating
Rho GTPases15,16,17. Previous studies have shown that Vilse in _Drosophila_ acts downstream of robo receptor through its WW domain to regulate Rac/cdc42-dependent cytoskeletal changes and
neurogenesis. Genetic interactions between Vilse, slit, and robo mutants claim that Vilse transduces signals downstream of Robo receptor to regulate Rac-dependent midline repulsion in
_Drosophila_15,16. In mice, Vilse interacts with connector enhancer of KSR-2 (CNK2) in the postsynaptic membrane also via WW domain to modulate Rac/Cdc42 signaling during spine morphogenesis
in hippocampal neurons17. Structurally, MyTH4 domain has a conserved helical bundle and is often located N-terminal to a FERM domain to associate with microfilaments and microtubules18.
However, there is no FERM domain in Vilse and the role of Vilse MyTH4 domain in regulating Rho activity and cytoskeleton remains to be elucidated. In this report, we characterized the
structural and functional phenotypes of VilseΔ/Δ mice with anatomical, physiological and behavioral means. In VilseΔ/Δ mice, the dendritic architecture in the hippocampal neurons were
altered while hippocampus-mediated spatial learning/memory function was impaired. Electrophysiological recordings in the hippocampal CA1 region also revealed defects in synaptic transmission
and plasticity in mutant mice. Our results led to a conclusion that Vilse is essential for embryonic development and required for dendritic structure and synaptic function. RESULTS
GENERATION OF _VILSE_ KNOCKOUT MICE To address the function of Vilse _in vivo_, embryonic stem (ES) cells with LacZ reporter insertion in Vilse allele were obtained from International Mouse
Phenotyping Consortium (IMPC). The fragment containing exons 1 to 4 of _Vilse_ is designated to be spliced to the lacZ trapping element in this targeting cassette (Fig. 1a). Chimeras capable
of germ-line transmission were backcrossed to C57BL/6 mice to generate _Vilse_ heterozygous mice (_Vilse_+/−). Male and female _Vilse_+/− mice were intercrossed to produce _Vilse_
homozygous mice (_Vilse_−/−). Of 102 progeny analyzed, _Vilse_+/+ and _Vilse_+/− mice were approximately in a ratio of 1:2 without noticeably phenotypic differences (data not shown).
However, _Vilse_−/− mice died at early embryonic stages. Dead embryos could be found at E13.5 with two interrupted alleles (Fig. 1b), indicating that deficiency of _Vilse_ might result in
prematurely embryonic death. Whole embryo extracts were collected and immunoblotting analysis revealed the loss of Vilse protein in _Vilse_−/− embryos at E10.5 (Fig. 1c). We further isolated
embryos at E9.0 for H&E staining. At this stage, prospective lungs, brain, forelimb and hindlimb buds appeared in _Vilse_+/+ and _Vilse_+/− embryos. By contrast, _Vilse_−/− embryos did
not display normal organogenesis, which had a severely impaired embryo morphology (Fig. 1d), indicating that the ablation of Vilse deteriorated the development and survival of _Vilse_−/−
embryos Since Vilse is important for cell viability, we set out to generate Vilse’s conditional knockout mice. To determine which Cre-transgenic mice could be applied for Vilse ablation, we
examined the expression of Vilse in different tissues. The exons 1–4 of Vilse are expected to be fused to β-galactosidase in the original gene-trap construct (Fig. 1a). Therefore, the
Vilse-LacZ fusion protein could be visualized by the addition of X-gal as substrates. The appearance of embryos at E13.5 displayed blue colors with ubiquitously spatial patterns (Fig. 1e).
Immunoblotting analysis also revealed a ubiquitous expression of Vilse in a variety of adult tissues. More interestingly, the expression of Vilse in the brain is much higher than other
tissues (Fig. 1f). The double labeling experiment revealed that Vilse predominantly colocalized with MAP2 in the pyramidal cells of hippocampal CA1 neurons. By contrast, little
colocalization of Vilse with Iba-1, a microglia marker, and glial fibrillary acidic protein (GFAP), an astrocyte marker, were found in the hippocampal CA1 region, indicating that Vilse
mainly expressed in CA1 neurons but not in glia cells. We therefore included Camk2a-driven Cre expression strategy to generate the forebrain-specific conditional knockout of Vilse to explore
the function of Vilse in the brain. GENERATION OF VILSEΔ/Δ MICE BY CAMK2A-CRE MEDIATED RECOMBINATION There are two _FRT_ sequences flanking the gene-trap LacZ cassette. This LacZ cassette
could be removed by FLP recombinase to leave exon 5 (a.a. 197–648) flanked by two loxP sequences (Fig. 2a), which can be further eliminated by Cre recombinase to produce VilseΔ/Δ mice.
Therefore, _Vilse_+/− mice were first mated with _FLP_ transgenic mice to produce Vilse_fl/+_ and Vilse_fl/fl_ littermates. Both alleles containing lacZ cassettes were successfully removed
by FLP recombinase shown as fl/fl (Fig. 2b). These Vilsefl/fl pups were viable, supporting the notion that ablation of Vilse resulted in embryonic lethality. Subsequently, Vilsefl/fl mice
were crossed with Camk2a-Cre transgenic mice to produce VilseΔ/Δ mice. VilseΔ/Δ mice were viable with no noticeably developmental defects. Immunohistochemistry of adult VilseΔ/Δ mice
confirmed a significant reduction of Vilse in the cerebral cortex and hippocampus (Fig. 2c) and immunoblotting analysis revealed the loss of Vilse in the hippocampal extracts (Fig. 2d).
DENDRITIC STRUCTURES OF CA1 PYRAMIDAL NEURONS WERE ALTERED IN VILSEΔ/Δ MICE To estimate the synaptic function in the structural aspect, we examined the dendritic architecture of CA1 neurons.
Golgi-Cox impregnated CA1 pyramidal neurons (30 neurons from control and 30 neurons from VilseΔ/Δ mice) were reconstructed and analyzed using Neurolucida software (Fig. 3a). The dendritic
complexity was evaluated using the concentric-ring method of Sholl in a three-dimensional manner. Compared with controls, the numbers of intersections between dendritic branches and the
concentric balls were significantly reduced in both apical and basilar dendrites of CA1 neurons in VilseΔ/Δ mice (Fig. 3b). The numbers of dendritic segments (Fig. 3c), bifurcation nodes and
terminal ends (Fig. 3e) were also reduced, especially in the apical dendrites of CA1 neurons in VilseΔ/Δ mice. These results indicated a reduction in dendritic complexity in mutant mice. We
further analyzed the length of dendritic segments. Compared with those in controls, the length between two bifurcation nodes (intermodal length) was shorter in the basilar dendrites of CA1
neurons in mutants, while the length of terminal segments was reduced in both apical and basilar dendrites of these cells (Fig. 3d), leading to the shorter dendritic length in VilseΔ/Δ mice
(Fig. 3e). These results revealed that the elongation and bifurcation of dendrites are affected in the absence of Vilse. We also characterized the density of dendritic spines, the
protrusions on the dendrites where the excitatory synapses reside. Interestingly, CA1 pyramidal neurons in VilseΔ/Δ mice had greater spine density in both apical and basilar dendrites than
those in control mice (Fig. 3f). We further examined the features of dendritic spines (Fig. 3g). Compared with those in control mice, greater portion of immature spine (thin spines) (Fig.
3h) and longer spine length (Fig. 3i) was noticed in the CA1 pyramidal neurons of VilseΔ/Δ mice (2094 spines counted in control mice and 2681 in VilseΔ/Δ mice). Additionally, we examined the
dendritic architecture of granule cells in the dentate gyrus (DG). Similarly, reduced dendritic length and complexity and greater spine density were found in DG cells of VilseΔ/Δ mice
(Supplementary Fig. 1). DG granule cells in VilseΔ/Δ mice also had more immature spines compared with those in control mice (2834 protrusions counted in control group and 2302 in VilseΔ/Δ
mice, Supplementary Fig. 1g). Together, the morphological alterations of hippocampal neurons in the absence of Vilse suggested the changes of hippocampal function in VilseΔ/Δ mice. SPATIAL
MEMORY IMPAIRED IN VILSEΔ/Δ MICE To evaluate the function of the hippocampus in VilseΔ/Δ mice, hippocampus-mediated spatial learning/memory tests were performed. In training phase of Morris
water maze test, VilseΔ/Δ and control mice behaved similarly in finding the hidden platform on Day 1, suggesting the comparable visual and mobile capabilities among control and VilseΔ/Δ
mice. However, during day 2 to day 5, a remarkably longer latency to find the hidden platform was exhibited in VilseΔ/Δ mice compared with control groups, suggesting a poorer spatial
learning ability in VilseΔ/Δ mice (Fig. 4a). To evaluate the spatial memory of mice, a probe test was performed on day 6 in which the hidden platform was removed and swim paths of mice were
traced. As anticipated, control mice swam to the removed platform areas faster than VilseΔ/Δ mice did (Supplementary Fig. S2). Male and female mice displayed similar patterns (Supplementary
Fig. S2), indicating that gender differences in VilseΔ/Δ mice were not associated with impaired spatial memory. Furthermore, in the Y-maze test, VilseΔ/Δ mice also displayed a defective
spatial working memory compared with control mice (Fig. 4b). However, the fear memory measured by passive avoidance test did not imply a significant difference between control and VilseΔ/Δ
mice (Fig. 4c). Together, these results indicated the hippocampus-mediated spatial learning/memory function is impaired in VilseΔ/Δ mice. REDUCED LONG-TERM POTENTIATION IN HIPPOCAMPAL CA1
AREA OF VILSEΔ/Δ MICE To bridge the structural defects in hippocampal neurons and impaired hippocampal function in VilseΔ/Δ mice, the features of synaptic transmission in the hippocampal CA1
neurons were examined. We recorded field excitatory postsynaptic potential (fEPSP) in hippocampal CA1 slices taken from control and VilseΔ/Δ mice. Input-output curves showed that the slope
of the field excitatory postsynaptic potential (fEPSP) was significantly lowered in VilseΔ/Δ mice compared with control mice as the stimulus intensity increased (5–7 V, P = 0.002; 8–10 V, P
< 0.001) (Fig. 5a), reflecting a dampened basal synaptic transmission in VilseΔ/Δ mice. We next measured the responses to paired-pulse stimuli. Paired-pulse facilitation (PPF) is a
character at the Schaffer collateral-CA1 synapse and considered as a form of short-term synaptic plasticity in which the second response to the closely spaced paired stimuli is increased due
to residual Ca2+ in the presynaptic nerve terminal from the first stimulus19. No difference in the PPF between the control and VilseΔ/Δ mice was noticed, indicating that Vilse deficiency
did not affect short-term presynaptic plasticity (Fig. 5b). Long-term potentiation (LTP), a use-dependent change in synaptic strength, has been well established as a cellular substrate for
information storage in the brain20. We compared the properties of LTP of fEPSP in hippocampal CA1. After a period of at least 10 min baseline fEPSP recording, application of high
frequency-stimulation immediately induced a post-tetanus potentiation (PTP), followed by a long-term potentiation (LTP) of fEPSP in slices. In wild-type mice, the PTP and LTP were 233 ± 27%
and 161 ± 8% of the baseline, respectively. In contrast, the PTP and LTP were 175 ± 12% (p < 0.05, paired _t_ test) and 91 ± 8% of the baseline (p = 0.450, paired t-test), respectively.
The significant difference between control and VilseΔ/Δ mice was found in LTP (p < 0.01, Mann-Whitney _U_-test) but not in PTP (p = 0.073, Mann-Whitney _U_-test), indicating a deficit in
long-term synaptic plasticity in VilseΔ/Δ mice (Fig. 5c). DISCUSSION To avoid the premature lethality, forebrain neuron-specific conditional Vilse knockout mice were generated.
Camk2a-mediated Vilse ablation in mice did not result in premature embryonic death, suggesting that Vilse might not be essential for cell viability in neuronal cells. We then evaluated the
consequences of Vilse knockout in the aspects of neuronal morphology and synaptic transmission in the hippocampal neurons and hippocampus-mediated spatial learning and memory function.
Results from water maze and Y-maze tests revealed the defective spatial memory in VilseΔ/Δ mice. Defective spatial memory in mutant mice could be reasoned by reduced synaptic transmission
and impaired synaptic plasticity such as LTP as well as the morphological alterations in dendritic complexity and spine properties in hippocampal CA1 neurons. The structural and functional
changes of neurons in VilseΔ/Δ mice are likely due to the dysregulation of actin cytoskeletons resulted from the changes of Rho GTPases downstream of Vilse. Rho GTPases not only regulate
actin filament reorganization, but also participate in CTL- and Fas-induced apoptosis. Dominant-negative mutants of Rho GTPases and _Clostridium difficile_ toxin B, an inhibitor of all Rho
GTPases, inhibit cellular susceptibility to Cytotoxic T lymphocyte- and Fas-induced apoptosis21. Furthermore, Rho GTPase signaling pathways has been shown to be associated with apoptosis and
the killing of superfluous cells is important during embryonic development22, indicating that loss of Vilse may potentiate the over-activation of Rho GTPases in embryos and consequently
result in embryonic lethality. Intriguingly, Camk2a-mediated Vilse ablation in the forebrain did not result in a significant increment of RhoA GTPase activity (data not shown), suggesting
that the loss of Vilse might be compensated by other RhoGAPs to modulate RhoA GTPase activity. It is always a contention that there are approximately 70 RhoGAPs, from yeast to human, in
contrast to 22 Rho proteins3. One plausible explanation is that some GAPs have preferential tissue expression and tissue specific functions. Their specific spatial and temporal expressions
during development enable them to be tightly coped with Rho GTPases and modulate synaptic function through the interaction with diverse effectors23. Several GAPs have been shown to uniquely
regulate synaptic development and plasticity24,25,26. We used forebrain-specific Vilse conditional knockout mice model to demonstrate that Vilse is involved in the modulation of dendritic
architecture including dendritic length, branching property as well as the density, shape and length of dendritic spines. In CA1 and DG neurons of VilseΔ/Δ mice, greater spine density was
noticed. It seemed odd to correlate this result with the reduced synaptic transmission. Since the profiles of dendritic spines are important for the transmission and integration of neural
signals27, altered dendritic architectures in CA1 and DG cells of VilseΔ/Δ mice may result in defects of the synaptic transmission and integration of neural information. Compared with the
controls, greater amounts of immature and unstable spines were observed in CA1 and DG neurons of VilseΔ/Δ mice, in line with the _in vitro_ study of interfering the binding between Vilse and
CNK217. Removal of Vilse would affect the structural integrity of postsynaptic scaffold which might in turn influence the distribution and function of postsynaptic receptors and ion
channels and result in impaired synaptic transmission. The increased density of dendritic spines could be a compensatory change. Alternatively, these excess dendritic spines might be spared
from synaptic activity-mediated spine pruning. Indeed, this notion is partly supported by electrophysiological examinations on basal synaptic transmission and long term potentiation. Little
change in paired pulse facilitation suggests that short-term presynaptic plasticity at CA1 synapse is not altered in VilseΔ/Δ mice. Nevertheless, the capability for CA1 synapses to undergo
the use-dependent change is limited in VilseΔ/Δ mice as LTP expression is impaired. Since the hippocampus is specific to spatial memory, these observations, including dendritic architecture
and electrophysiological examinations, echoed the results of water maze and Y maze tests and provide evidence that Vilse is an important player in synaptic plasticity for spatial memory.
Together, the structural changes of dendritic architecture and disruption of postsynaptic integrity might account for the hippocampal LTP deficiency and behavioral deficits in
hippocampus-related learning and memory tasks in Vilse conditional KO mice. METHODS ANTIBODIE Mouse anti-Vilse monoclonal antibody was raised against 6xHis-tagged Vilse recombinant protein
(a.a. 900–1114). Rabbit anti-MAP2 antibody was obtained from Millipore (Temecula, CA). Goat anti-Iba-1 antibody was from GeneTex (Hsinchu, Taiwan). Rabbit anti-GFAP antibody was from DAKO
(Midland, Canada). Mouse anti-α-tubulin antibody was from Sigma-Aldrich (St. Louis, MI). (Irvine, CA). WHOLE CELL EXTRACTS Whole cell extracts were prepared with RIPA lysis buffer. To
prepare cytosolic and nuclear extracts, 1 × 108 cells were lysed in buffer A (10 mM HEPES (pH 7.9), 10 mM KCl, 1.5 mM MgCl2, 0.34 M sucrose, 10% glycerol, 1 mM DTT, 0.1% Triton X-100,
protease inhibitors). After incubation at 37°C for 5 min, nuclei were lysed in 1 mL of buffer B (3 mM EDTA, 0.2 mM EGTA, 1 mM DTT, protease inhibitors). Insoluble chromatin was separated via
centrifugation (5 min, 2,500 rpm) and resuspended in 0.5 mL Laemmli buffer and then sonicated for 5 min for SDS-PAGE. IMMUNOFLUORESCENCE Mice were perfused with PBS followed by
paraforaformaldehyde fixation. The fixed brains were cryoprotected in 30% sucrose and cut to 30 mm thick sections. The sections were blocked in 10% normal goat serum and 2% bovine serum
albumin in PBST (phosphate-buffered saline and 2% Triton X-100) and incubated with individual primary antibodies against Vilse, MAP2, Iba-1, and GFAP in blocking buffer overnight.
Subsequently, DyLight 488- and Alexa fluor 594-conjugated secondary antibodies (Jackson ImmunoResearch Laboratories, West Grove, PA) were applied. Sections were coverslipped with antifade
mounting medium (Southern Biotech, Birmingham, AL) and examined on a confocal microscope (Leica TCS SP5, Wetzlar, Germany). GENERATION OF VILSE CONDITIONAL KNOCKOUT MICE All animal studies
were performed in compliance with the guidelines of the Institutional Animal Use and Care Committee, Academia Sinica. An ES cell containing LacZ reporter-tagged insertion in Vilse/Arhgap39
allele were obtained from International Mouse Phenotyping Consortium (IMPC) using a combination of both a reporter-tagged and a conditional mutation28. Based on the original construct, exon1
to exon4 of _Vilse_ is spliced to a lacZ trapping element in the targeting cassette containing the mouse En2 splice acceptor and the SV40 polyadenylation sequences19. This ES cell was
injected into C57BL/6 blastocysts to generate chimeric mice. Germ-line transmission of the mutant allele was tested by PCR while two independent male chimeric mice were back-crossed with
female C57BL/6 mice to generate Vilse heterozygous F1 offsprings. Conditional alleles were further generated by removal of the gene-trap LacZ cassette by FLP recombinase, which reverts the
mutation to wild type allele, but leaving loxP sites on either side of exon 5 (a.a 197–648). Floxed homozygous male and female mice (Vilsefl/fl) were mated with the mouse
calcium/calmodulin-dependent protein kinase II alpha promoter driving Cre recombinase transgenic mice (Camk2a-Cre mice, a gift from Dr. Che-Kun Shen, Academia Sinica) to produce VilseΔ/Δ
mice. All animal studies were performed in compliance with the guidelines of the Institutional Animal Use and Care Committee, Academia Sinica. GENOTYPIN Mouse tail DNAs were isolated using
EasyPure Genomic DNA spin kit (Bioman, Taipei, Taiwan) according to manufacturer’s instructions. The following pairs of primers were used, FRT1, 5′-TACTACGTGCACTCAGGTGATCCT-3′. LoxP6,
5′-AGCAGGTCACAAATGTCACTCTGC-3′. Camk2aF1, 5′-GGTTCTCCGTTTGCACTCAG-3′. iCreR1, 5′-TCCCTCACATCCTCAGGTTC-3′ FRT1 and LoxP6 were used to amplify the wild-type and floxed Vilse allele with
products of 380 and 570 bp, respectively. Camk2aF1 and iCreR1 were used to amplify the presence of Camk2a-iCre with a product of 380 bp. PCR reaction was performed at 95 °C for 7 min
followed by 30 cycles of 95 °C for 20 s, 53 °C for 30 s, and 72 °C for 60 s. GOLGI-COX IMPREGNATION AND MORPHOMETRIC ANALYSES After transcardiac perfusion with phosphate-buffered saline and
fixative (4% paraformaldehyde in phosphate buffer, pH 7.4), whole brains were taken and immersed in the impregnation solution from the FD Rapid Golgi Stain kit (NeuroTechnologies, Ellicott
City, MD) for Golgi-Cox impregnation as previously described29. In brief, after 3 weeks of impregnation, brain samples were sectioned at a thickness of 150 μm and incubated with a mixture of
developer and fixer solutions (FD Rapid Golgi Stain kit), washed and mounted. The pyramidal neurons in the hippocampal CA1 region and granule cells in the dentate gyrus (DG) were examined
under a light microscope with a 20x objective lens for dendritic morphology and 100x objective lens for spine analysis, respectively. Series of pictures were taken by a CCD camera with the
aid of computer-controlled motorized stage using the Stereo Investigator system (MBF Bioscience, Williston, VT). The morphology of selected neurons was reconstructed and analyzed with
Neurolucida software (MBF Bioscience). Data were expressed as the mean ± SEM. Two-tailed unpaired Student’s t-test was used for statistical analysis. MORRIS WATER MAZE All experiments
regarding behavior tests, Golgi stain, and electrophysiology were performed in accordance with guidelines approved by National Taiwan University College of Medicine and College of Public
Health (20150132). Mice were reared in the animal facility of National Taiwan University under a 12-h light/dark cycle with free access to food and water. The properties of
hippocampus-mediated spatial learning and memory were evaluated using Morris water maze test30 with minor modifications. Briefly, the circular pool was 1.88 m in diameter and contained water
(temperature 19 °C) that was made opaque with non-fat milk. A hidden platform was put in a fixed location 1 cm below the water surface. During the training period, individual mouse was
placed in the water facing the pool wall at one of four start points (north, south, east, or west). Upon release into the water, the mouse was allowed to swim for 90 secs to locate the
hidden platform. An additional 30 secs were then allowed for mice to stand still on the platform and escape from the water before being removed. If the mouse failed to stand on the platform,
it was guided to the platform and remained there for 30 sec. Four trials of different start points were given each day for 5 consecutive days. After 5-day training period, another test
trial was given in which the platform was removed. Latencies to reach the hidden platform and the swim paths were recorded with an automatic video tracking system. Y-MAZE TEST Mice were
placed individually at the end of one arm of the Y-maze and allowed to move within three arms freely for an 8-min period. The total number and series of arm entries were recorded. The number
of non-overlapping entrance sequences (e.g. ABC, BCA) was defined as the number of alternations. The spontaneous alternation (%) was calculated as: (number of alternations)/ (total number
of arm entries-2) × 10031. PASSIVE AVOIDANCE TES During habituation, mice were placed into the light side of the chamber and the latency to enter the dark side was recorded. On the training
day, a mild foot shock is delivered upon entry to the dark side of the chamber. Memory of passive avoidance was assessed at 24 h and 48 h after training and the latency to enter the dark
side was analyzed. ELECTROPHYSIOLOGY VilseΔ/Δ or control mice aged 8 weeks were decapitated and their brains were quickly removed and placed in ice-cold artificial cerebrospinal fluid (ACSF)
containing (in mM) NaCl, 119; KCl, 2.5; NaHCO3, 26.2; NaH2PO4, 1; MgSO4, 1.3; CaCl2, 2.5; glucose, 11; pH was adjusted to 7.4 by gassing with 5% CO2 : 95% O2. Transverse hippocampal slices
of 450 μm thickness were cut with a vibrating tissue slicer (Dosaka microslicer, Japan) and transferred to an interface-type holding chamber at room temperature (25 °C). For extracellular
field potential recording, slices were transferred to an immersion-type recording chamber, superfused with ACSF containing 0.1 mM picrotoxin at room temperature. The superfusion rate was
controlled at 2 mL/min. To prevent epileptiform discharge of pyramidal neurons, a surgical cut was made at the border between areas CA1 and CA3. A glass pipette filled with 3 M NaCl was
positioned at stratum radiatum of CA1 to record field excitatory postsynaptic potentials (fEPSP) evoked by a bipolar stainless steel electrode (FHC, Bowdoinham, ME) placed in the vicinity of
recording pipette. To stimulate Schaffer collateral branches, a constant current pulse of 0.5 ms duration (DS3, Digitimer, UK) was delivered through the stimulating electrodes every 30 s
with the intensity adjusted so that 40 ± 50% of the maximal response was elicited. Long-term potentiation (LTP) of fEPSP was induced by high-frequency-stimulation consisting of 3 trains of
100 pulses at 100 Hz with inter-train interval being 10 s. All signals were filtered at 2 kHz by a low-pass Bessel filter provided by the amplifier (Axopatch-1D, Axon Instruments, Foster
City, CA) and digitized at 5 kHz using CED micro 1401 interface running Signal software provided by CED (Cambridge Electronic Design, Cambridge, UK). The initial slope of fEPSP was measured
for data analysis. Synaptic responses were normalized to average values measured over a baseline period. The averaged slope of fEPSPs recorded between 0–2 and 55–60 min after high frequency
stimulation was used for statistical comparisons of post-tetanus potentiation (PTP) and LTP, respectively. All data given were Mean ± SEM, and were statistically compared using either paired
t-tests or one-way ANOVA. The criterion for significance was P < 0.05. ADDITIONAL INFORMATION HOW TO CITE THIS ARTICLE: Lee, J.-Y. _et al_. Important roles of Vilse in dendritic
architecture and synaptic plasticity. _Sci. Rep._ 7, 45646; doi: 10.1038/srep45646 (2017). PUBLISHER'S NOTE: Springer Nature remains neutral with regard to jurisdictional claims in
published maps and institutional affiliations. REFERENCES * Ridley, A. J. Life at the leading edge. _Cell_ 145, 1012–1022 (2011). Article CAS Google Scholar * Olofsson, B. Rho guanine
dissociation inhibitors: pivotal molecules in cellular signalling. _Cell. Signal._ 11, 545–554 (1999). Article CAS Google Scholar * Tcherkezian, J. & Lamarche-Vane, N. Current
knowledge of the large RhoGAP family of proteins. _Biol. Cell_ 99, 67–86 (2007). Article CAS Google Scholar * Bourne, J. N. & Harris, K. M. Balancing structure and function at
hippocampal dendritic spines. _Annu. Rev. Neurosci._ 31, 47–67 (2008). Article CAS Google Scholar * Luo, L. Rho GTPases in neuronal morphogenesis. _Nat. Rev. Neurosci._ 1, 173–180 (2000).
Article CAS Google Scholar * Ahnert-Hilger, G. et al. Differential effects of Rho GTPases on axonal and dendritic development in hippocampal neurones. _J. Neurochem_. 90, 9–18, (2004).
Article CAS Google Scholar * Newey, S. E., Velamoor, V., Govek, E. E. & Van Aelst, L. Rho GTPases, dendritic structure, and mental retardation. _J. Neurobiol._ 64, 58–74 (2005).
Article CAS Google Scholar * Duman, J. G., Mulherkar, S., Tu, Y. K., J, X. C. & Tolias, K. F. Mechanisms for spatiotemporal regulation of Rho-GTPase signaling at synapses. _Neurosci.
Lett._ 601, 4–10 (2015). Article CAS Google Scholar * Burridge, K. & Wennerberg, K. Rho and Rac take center stage. _Cell_ 116, 167–179 (2004). Article CAS Google Scholar * Scott,
E. K., Reuter, J. E. & Luo, L. Small GTPase Cdc42 is required for multiple aspects of dendritic morphogenesis. _J. Neurosci._ 23, 3118–3123 (2003). Article CAS Google Scholar *
Nakayama, A. Y. & Harms, M. B. & Luo, L. Small GTPases Rac and Rho in the maintenance of dendritic spines and branches in hippocampal pyramidal neurons. _J. Neurosci._ 20, 5329–5338
(2000). Article CAS Google Scholar * Kim, I. H., Wang, H., Soderling, S. H. & Yasuda, R. Loss of Cdc42 leads to defects in synaptic plasticity and remote memory recall. _Elife_ 3
(2014). * Haditsch, U. et al. A central role for the small GTPase Rac1 in hippocampal plasticity and spatial learning and memory. _Mol. Cell. Neurosci._ 41, 409–419 (2009). Article CAS
Google Scholar * Tashiro, A., Minden, A. & Yuste, R. Regulation of dendritic spine morphology by the rho family of small GTPases: antagonistic roles of Rac and Rho. _Cereb. Cortex_ 10,
927–938 (2000). Article CAS Google Scholar * Lundstrom, A. et al. Vilse, a conserved Rac/Cdc42 GAP mediating Robo repulsion in tracheal cells and axons. _Genes Dev._ 18, 2161–2171 (2004).
Article Google Scholar * Hu, H. et al. Cross GTPase-activating protein (CrossGAP)/Vilse links the Roundabout receptor to Rac to regulate midline repulsion. _Proc. Natl. Acad. Sci. USA._
102, 4613–4618 (2005). Article ADS CAS Google Scholar * Lim, J., Ritt, D. A., Zhou, M. & Morrison, D. K. The CNK2 scaffold interacts with vilse and modulates Rac cycling during spine
morphogenesis in hippocampal neurons. _Curr. Biol._ 24, 786–792 (2014). Article CAS Google Scholar * Kerber, M. L. & Cheney, R. E. Myosin-X: a MyTH-FERM myosin at the tips of
filopodia. _J. Cell Sci._ 124, 3733–3741 (2011). Article Google Scholar * Katz, B. & Miledi, R. A study of synaptic transmission in the absence of nerve impulses. _J. Physiol_. 192,
407–436 (1967). Article CAS Google Scholar * Lynch, M. A. Long-term potentiation and memory. _Physiol. Rev._ 84, 87–136, doi: 10.1152/physrev.00014.2003 (2004). Article CAS PubMed
Google Scholar * Subauste, M. C. et al. Rho family proteins modulate rapid apoptosis induced by cytotoxic T lymphocytes and Fas. _J. Biol. Chem_. 275, 9725–9733 (2000). Article CAS Google
Scholar * Coleman, M. L. & Olson, M. F. Rho GTPase signalling pathways in the morphological changes associated with apoptosis. _Cell Death Differ_. 9, 493–504 (2002). Article CAS
Google Scholar * Tolias, K. F., Duman, J. G. & Um, K. Control of synapse development and plasticity by Rho GTPase regulatory proteins. _Prog. Neurobiol._ 94, 133–148, (2011). Article
CAS Google Scholar * Ba, W. et al. ARHGAP12 Functions as a Developmental Brake on Excitatory Synapse Function. _Cell Rep_ 14, 1355–1368 (2016). Article CAS Google Scholar * Guerrier, S.
et al. The F-BAR domain of srGAP2 induces membrane protrusions required for neuronal migration and morphogenesis. _Cell_ 138, 990–1004 (2009). Article CAS Google Scholar * Ip, J. P. et
al. alpha2-chimaerin controls neuronal migration and functioning of the cerebral cortex through CRMP-2. _Nat. Neurosci._ 15, 39–47 (2011). Article Google Scholar * Parekh, R. & Ascoli,
G. A. Quantitative investigations of axonal and dendritic arbors: development, structure, function, and pathology. _Neuroscientist_ 21, 241–254 (2015). Article CAS Google Scholar *
Skarnes, W. C. et al. A conditional knockout resource for the genome-wide study of mouse gene function. _Nature_ 474, 337–342 (2011). Article CAS Google Scholar * Li, W. Y., Chang, Y. C.,
Lee, L. J. & Lee, L. J. Prenatal infection affects the neuronal architecture and cognitive function in adult mice.. _Dev. Neurosci._ 36, 359–370 (2014). Article Google Scholar *
Vorhees, C. V. & Williams, M. T. Morris water maze: procedures for assessing spatial and related forms of learning and memory. _Nat. Protoc._ 1, 848–858, (2006). Article Google Scholar
* Kozlov, A. P., Druzin, M. Y., Kurzina, N. P. & Malinina, E. P The role of D1-dependent dopaminergic mechanisms of the frontal cortex in delayed responding in rats. _Neurosci. Behav.
Physiol._ 31, 405–411 (2001). Article CAS Google Scholar Download references ACKNOWLEDGEMENTS We thank Transgenic Core Facility at National Taiwan University Hospital to generate chimeric
mice. This work was supported by Ministry of Science and Technology (MOST 103-2311-B-002-023) and National Taiwan University (103R7602B3) to MSC and in part supported by a grant from MOST
to LJL. AUTHOR INFORMATION Author notes * Jin-Yu Lee and Li-Jen Lee: These authors contributed equally to this work. AUTHORS AND AFFILIATIONS * Institute of Biochemical Sciences, College of
Life Science, National Taiwan University, Taipei, Taiwan Jin-Yu Lee, Hsin-An Shih & Mau-Sun Chang * Graduate Institute of Anatomy and Cell Biology, National Taiwan University, Taipei,
Taiwan Li-Jen Lee & Ho-Ching Chang * Institute of Brain and Mind Sciences National Taiwan University, Taipei, Taiwan Li-Jen Lee * Neurobiology and Cognitive Science Center, National
Taiwan University, Taipei, Taiwan Li-Jen Lee * Department of Superintendent Office, Mackay Memorial Hospital, Taipei, Taiwan Chih-Chen Fan * Department of Medical Laboratory Science and
Biotechnology, Yuanpei University, Hsinchu, Taiwan Chih-Chen Fan * Department of Medical Technology, Jen-Teh Junior College of Medicine, Nursing and Management, Miaoli, Taiwan Chih-Chen Fan
* Department of Life Science, College of Life Science, National Taiwan University, Taipei, Taiwan Ming-Yuan Min * Institute of Biological Chemistry, Academia Sinica, Taipei, Taiwan Mau-Sun
Chang Authors * Jin-Yu Lee View author publications You can also search for this author inPubMed Google Scholar * Li-Jen Lee View author publications You can also search for this author
inPubMed Google Scholar * Chih-Chen Fan View author publications You can also search for this author inPubMed Google Scholar * Ho-Ching Chang View author publications You can also search for
this author inPubMed Google Scholar * Hsin-An Shih View author publications You can also search for this author inPubMed Google Scholar * Ming-Yuan Min View author publications You can also
search for this author inPubMed Google Scholar * Mau-Sun Chang View author publications You can also search for this author inPubMed Google Scholar CONTRIBUTIONS J.Y.L. and L.J.L.
contributed equally to this work. J.Y.L. performed immunofluorescence, immunoblotting, and genotyping. L.J.L. and H.C.C. performed Golgi staining, immunofluorescence, and data analysis.
C.C.F. conducted immunocytochemistry. H.A.S. assisted I.F. and I.B. M.Y.M. carried out electrophysiology experiments. L.J.L./M.Y.M./M.S.C. wrote this manuscript. M.S.C. supervised this
project. CORRESPONDING AUTHOR Correspondence to Mau-Sun Chang. ETHICS DECLARATIONS COMPETING INTERESTS The authors declare no competing financial interests. SUPPLEMENTARY INFORMATION
SUPPLEMENTARY INFORMATION (DOC 2057 KB) RIGHTS AND PERMISSIONS This work is licensed under a Creative Commons Attribution 4.0 International License. The images or other third party material
in this article are included in the article’s Creative Commons license, unless indicated otherwise in the credit line; if the material is not included under the Creative Commons license,
users will need to obtain permission from the license holder to reproduce the material. To view a copy of this license, visit http://creativecommons.org/licenses/by/4.0/ Reprints and
permissions ABOUT THIS ARTICLE CITE THIS ARTICLE Lee, JY., Lee, LJ., Fan, CC. _et al._ Important roles of Vilse in dendritic architecture and synaptic plasticity. _Sci Rep_ 7, 45646 (2017).
https://doi.org/10.1038/srep45646 Download citation * Received: 02 November 2016 * Accepted: 28 February 2017 * Published: 03 April 2017 * DOI: https://doi.org/10.1038/srep45646 SHARE THIS
ARTICLE Anyone you share the following link with will be able to read this content: Get shareable link Sorry, a shareable link is not currently available for this article. Copy to clipboard
Provided by the Springer Nature SharedIt content-sharing initiative