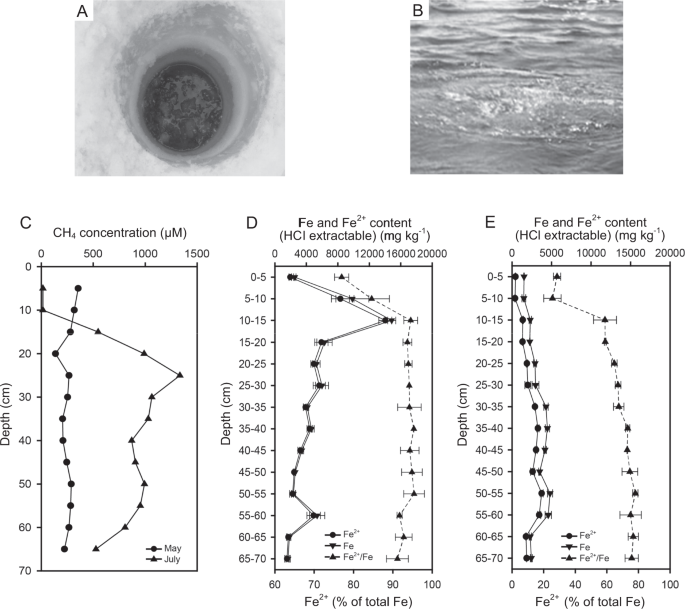
Metabolic flexibility of aerobic methanotrophs under anoxic conditions in arctic lake sediments
- Select a language for the TTS:
- UK English Female
- UK English Male
- US English Female
- US English Male
- Australian Female
- Australian Male
- Language selected: (auto detect) - EN
Play all audios:

ABSTRACT Methane (CH4) emissions from Arctic lakes are a large and growing source of greenhouse gas to the atmosphere with critical implications for global climate. Because Arctic lakes are
ice covered for much of the year, understanding the metabolic flexibility of methanotrophs under anoxic conditions would aid in characterizing the mechanisms responsible for limiting CH4
emissions from high-latitude regions. Using sediments from an active CH4 seep in Lake Qalluuraq, Alaska, we conducted DNA-based stable isotope probing (SIP) in anoxic mesocosms and found
that aerobic Gammaproteobacterial methanotrophs dominated in assimilating CH4. Aerobic methanotrophs were also detected down to 70 cm deep in sediments at the seep site, where anoxic
conditions persist. Metagenomic analyses of the heavy DNA from 13CH4-SIP incubations showed that these aerobic methanotrophs had the capacity to generate intermediates such as methanol,
formaldehyde, and formate from CH4 oxidation and to oxidize formaldehyde in the tetrahydromethanopterin (H4MPT)-dependent pathway under anoxic conditions. The high levels of Fe present in
sediments, combined with Fe and CH4 profiles in the persistent CH4 seep site, suggested that oxidation of CH4, or, more specifically, its intermediates such as methanol and formaldehyde
might be coupled to iron reduction. Aerobic methanotrophs also possessed genes associated with nitrogen and hydrogen metabolism, which might provide potentially alternative energy
conservation options under anoxic conditions. These results expand the known metabolic spectrum of aerobic methanotrophs under anoxic conditions and necessitate the re-assessment of the
mechanisms underlying CH4 oxidation in the Arctic, especially under lakes that experience extended O2 limitations during ice cover. You have full access to this article via your institution.
Download PDF SIMILAR CONTENT BEING VIEWED BY OTHERS MICROBIAL DRIVERS OF DMSO REDUCTION AND DMS-DEPENDENT METHANOGENESIS IN SALTMARSH SEDIMENTS Article Open access 25 October 2023
PHYLOGENETICALLY AND CATABOLICALLY DIVERSE DIAZOTROPHS RESIDE IN DEEP-SEA COLD SEEP SEDIMENTS Article Open access 19 August 2022 ACTIVE LITHOAUTOTROPHIC AND METHANE-OXIDIZING MICROBIAL
COMMUNITY IN AN ANOXIC, SUB-ZERO, AND HYPERSALINE HIGH ARCTIC SPRING Article 08 April 2022 INTRODUCTION Lakes at latitudes >50°N emit an estimated 18.8 Tg methane (CH4) per year directly
into the atmosphere [1], which is more than twice that from the oceans [2, 3]. Biological aerobic CH4 oxidation has been considered an important pathway to mitigate CH4 emission from Arctic
lakes, even at low temperatures (down to at least 4 °C) [4]. Arctic lakes are usually ice-covered for as much as 9 months from September through the following June [5]. When ice cover forms
on Arctic lakes, O2 concentration declines, with shallow lakes eventually becoming hypoxic (dissolved oxygen <1% equilibrium solubility). Dissolved CH4 accumulates in Arctic lake waters
as O2 dissipates [5]. Mechanisms and rates of CH4 oxidation under these hypoxic and anoxic conditions in Arctic lakes are not well documented, despite the fact that these low O2 conditions
persist for the majority of the year and any oxidation that may occur could impact the otherwise large net flux of CH4 [6, 7]. CH4 oxidation under anoxic conditions plays an important role
in controlling CH4 input to the atmosphere in various environments. In marine sediments, anaerobic oxidation of CH4 (AOM) generally occurs in the niches where CH4 and sulfate overlap [8].
However, nitrate, nitrite, iron III (Fe3+), and manganese IV (Mn4+) can also serve as electron acceptors for CH4 oxidation in the absence of O2 under anoxic conditions, especially in
freshwater environments where sulfate is less abundant than in seawater [9, 10]. Such reactions provide a greater free energy yield than sulfate-dependent CH4 oxidation under standard
conditions [9]. Decomposition products such as humic substances also can potentially act as an electron shuttle for AOM with metallic oxides as terminal electron acceptor in organic
carbon-rich environments [11]. AOM is thought to be performed mainly by anaerobic methane-oxidizing archaea (ANME), which are generally divided into three archaeal clades, including ANME-1
(with subgroups a and b) distantly related to _Methanomicrobiales_ and _Methanosarcinales_, ANME-2 (with subgroups a, b, and c) associated with the order _Methanosarcinales_, and ANME-3,
which is more related to _Methanococcoides_ spp. [8, 9]. ANME are commonly associated with sulfate-reducing bacteria (SRB) of the _Desulfosarcina/Desulfococcus_ branch of
_Deltaproteobacteria_. However, some ANME-2 also have been found to independently function in monospecific aggregations or as single filaments [12, 13]. “Omic” and single cell visualization
approaches showed that archaeal family _Methanoperedenaceae_ (formerly known as ANME-2d) can independently oxidize CH4 using nitrate as the terminal electron acceptor [14]. AOM coupled to
the reduction of Fe3+ and Mn4+ was first found to be associated with the groups _Bacteriodes_, _Proteobacteria_ (including _Geobacter_), _Acidobacteria_, and _Verrucomicrobia_, although
direct evidence was lacking [15]. Recently, members of _Methanoperedenaceae_ such as “_Candidatus_ Methanoperedens ferrireducens” and “_Candidatus_ Methanoperedens sp. MPEBLZ” have been
reported to be capable of coupling AOM to the reduction of Fe3+ and Mn4+ [15,16,17]. And, “_Candidatus_ Methylomirabilis oxyfera”, a member of the _Methylomirabilota_ phylum (NC10), has been
found to bypass the denitrification intermediate nitrous oxide by converting nitric oxide to dinitrogen and O2 under anoxic conditions, with O2 being utilized to aerobically oxidize CH4
[18]. Aerobic methanotrophs are curiously abundant in anoxic habitats [19,20,21], where they are generally thought to be dormant, existing as cysts or exospores, since O2 is required for
catalyzing the conversion of methane to methanol by either soluble or particulate methane monooxygenase (pMMO) [21]. Recently, however, aerobic methanotrophs have been reported to actively
oxidize CH4 under anoxic conditions. For example, Oswald et al. [22] found that Gammaproteobacterial methanotrophs dominated in the oxic/anoxic boundary and anoxic hypolimnion of Lake Zug
and CH4 oxidation was stimulated by addition of iron and manganese oxides under anoxic conditions. In sub-Arctic lake sediment, Martinez-Cruz et al. [19]. reported assimilation of
CH4-derived carbon by aerobic methanotrophs, methylotrophs, and iron reducers. Cabrol et al. [23]. reported that the aerobic methanotrophs _Methylomonadaceae_ (mainly _Methylobacter_) and
_Crenothrix_ actively oxidized CH4 in the anoxic water of Northwestern Siberian lakes in association with denitrifiers and iron-cycling partners. Milucka et al. [24]. found that aerobic CH4
oxidation coupled to oxygenic photosynthesis within the photic anoxic chemocline of Lake Cadagno. Additionally, aerobic methanotrophs have the metabolic flexibility to perform processes such
as fermentation or CH4 oxidation coupled to nitrate reduction to mitigate O2 starvation [25, 26]. Here we aimed to reveal the identities and metabolic pathways potentially utilized by
methanotrophs active under anoxic conditions in sediments obtained from an active CH4 seep in Lake Qalluuraq, an Arctic lake located near Atqasuk on the north slope of the Brooks Range in
Alaska. We applied molecular methodologies including DNA-based stable isotope probing (SIP) coupled with shotgun metagenomics and amplicon sequencing of bacterial and archaeal 16S rRNA genes
and quantitative real-time PCR (qPCR) with monitoring of geochemical parameters such as dissolved CH4 concentration and HCl-extractable Fe content. Our findings revealed that aerobic
methanotrophs have the ability to be active in anoxic environments where they have typically been presumed to be inactive and that they might couple the oxidation of CH4 or its intermediates
to the reduction of iron, which is abundant in the sediments of this lake. These observations have important implications for understanding the fate of CH4 in the anoxic sediments of Arctic
lakes and during the extended ice-covered season when more extensive lake anoxia develops. MATERIALS AND METHODS SAMPLING AND PHYSIO-CHEMICAL ANALYSIS Lake Qalluuraq, a tundra lake with a
persistent CH4 seep located near Atqasuk on the North Slope of the Brooks Range in Alaska, was sampled in May (ice-covered) and July (open-water), 2009. Ice thickness above the seep was ~50
cm in May. Sediments from the seep site (70°22.669′N, 157°20.925′W) were collected using coring tubes (7 cm diameter) and were subsampled within 12 h of collection. The sediment subsamples
were immediately placed into sealed plastic zipper freezer bags with air removed from the headspace. Samples were homogenized in the bag and subsamples were frozen at −80 °C for direct
molecular studies and Fe content analysis. The remaining sediment was stored at 4 °C and was used to conduct the SIP experiment immediately upon our return to the lab at the University of
Alaska Fairbanks. Dissolved CH4 concentrations in the sediment pore fluids were analyzed as described previously by He et al. [19]. Elemental and mineralogical composition of sediments was
estimated by X-ray diffraction analysis [27]. Since iron redox cycling exerts a wide-ranging influence on the biogeochemistry of sedimentary environments, we analyzed the HCl-extractable Fe
content including iron (II) (Fe2+), Fe3+, and total Fe [28]. In brief, ~10 g (wet weight) sediment subsample (stored frozen at −80 °C for 1 year after sampling) was acidified with HCl to pH
<1 and freeze-dried at −40 °C in the laboratory. Then, ~1 g freeze-dried sediment was taken for measurement of Fe2+ and total Fe content in an anaerobic chamber. Each sample was measured
in triplicate. The Fe2+ and total Fe content of the sediments were determined by the method described previously [29]. The resulting HCl-extractable Fe2+ to total Fe ratio of 90% indicated
that Fe oxidation during sample storage was negligible. SIP MICROCOSMS SIP microcosms were prepared by adding ~10 g (wet weight) of 25–50-cm-depth sediment to sterile 60-ml glass serum vials
in an anaerobic chamber, where the gas composition was 1–2% H2 and 98–99% N2. Five different treatments were established by adding combinations of 2-bromoethanesulfonic acid (BES, a
methanogen-specific inhibitor), sodium molybdate (SM, a specific inhibitor for sulfate reduction [30]), and Na2SO4 (EA, as a supplementary electron acceptor), and then were purged with
ultra-high-purity (UHP) N2 (99.999%) to remove O2. The five treatments were amended as follows, with treatment codes in parentheses: (1) BES and SM (BES+SM); (2) BES (BES); (3) SM (SM); (4)
EA (EA); and (5) unamended with BES, SM, or EA as control (None). Autoclaved O2−-free water was supplied at a total volume of 2 ml (including BES, SM, EA, and autoclaved water) for each
treatment. The final concentrations of BES, SM, and Na2SO4 were 10, 5, and 3 mM, respectively, in the treatments. Resazurin was added to a final concentration of 0.001% in the microcosms to
monitor for the presence of O2. The serum vials were flushed with UHP N2 and sealed with butyl rubber stoppers (Chemglass Life Sciences) in the anaerobic chamber containing 1–2% H2 and
98–99% N2, and pre-incubated for a month to exhaust any O2 potentially carried over from the original sediments. Then, the serum vials were flushed with UHP N2 and amended with BES, SM,
Na2SO4, autoclaved water, and resazurin and sealed with butyl rubber stoppers in the anaerobic chamber. The headspace of the serum vials was ~50 ml. 13CH4 (99 atom % 13C, Sigma-Aldrich, USA)
or CH4 (99.5% purity, as unlabeled control) was injected to obtain a headspace concentration of 5% CH4 (v/v), a concentration chosen to represent the extensive CH4 accumulation associated
with the active seep under ice-covered conditions. The serum vials were placed inside a cardboard box and incubated on a rotary platform shaker (100 rpm) in a cold room held at 10 °C. The
cold room was not illuminated other than by low-level incidental light entering through a small ~10 cm by 30 cm glass observation window in the door during the pre-incubation and SIP
experiment. Mesocosms experienced brief indoor light exposures during headspace gas sampling or exchange on the lab bench. All treatments were performed in triplicate. Headspace gas samples
(50 μl) were withdrawn from the microcosms at 20-d intervals using a syringe flushed with UHP N2 three times to remove trace O2 before drawing the samples. The CH4 concentration was
quantified as described previously [20]. CH4 oxidation potential was assessed from the zero-order decrease in CH4 concentration in the headspace of the serum vials during the incubation
period with differences analyzed statistically by ANOVA (one-way analysis of variance) using SPSS 19.0. Relatively stable δ13C of CH4 in the headspace indicated minimal CH4 production in the
vials. The serum vials were flushed with UHP N2 and sealed with butyl rubber stoppers in the anaerobic chamber and recharged with 13CH4 or CH4 every 40 or 80 days to remove 13CO2 and reduce
cross-feeding. The incubation was continued in the same manner until 240 days of incubation, when CH4 had been injected in the vials five times as described above. At the end of the
incubation, the sediment samples were harvested and frozen immediately at −80 °C. QUANTIFICATION OF 13C AND 18O IN HEADSPACE CO2 In order to monitor the conversion of 13CH4 and H218O into
CO2, we quantified the 13C and 18O in headspace CO2. Approximately 10 g (wet weight) of original sediment collected from the 25–50-cm depth range was added to sterile 60-ml glass serum vials
and incubated on a shaker as described in SIP microcosms. Considering the low CH4 oxidation activity of sediment in the first stage, 12CH4 was injected instead of 13CH4 in the first 40
days. Then the serum vials were flushed with UHP N2, amended with 10 μl H218O, and sealed with butyl rubber stoppers in the anaerobic chamber. 13CH4 was injected into the serum vials to the
initial concentrations of 5% (v/v). All serum vials were incubated as described above. One-milliliter headspace gas samples were periodically collected to measure the δ18O and δ13C values of
CO2 at the Alaska Stable Isotope Facility (University of Alaska Fairbanks) [31]. DNA EXTRACTION, GRADIENT CENTRIFUGATION, QPCR, AND T-RFLP ANALYSIS DNA was extracted from 3 to 4 g (wet
weight) of the sediment samples using the Bio101 Fast DNA Spin Kit for soil (MP Biomedicals, Solon, OH, USA). For SIP, equilibrium (isopycnic) density gradient centrifugation and
fractionation were performed as described previously [32]. The relative abundance of bacterial DNA in gradient fractions was determined by qPCR to identify the heavy (13C-labeled) DNA as
described by Leigh et al. [33]. The fractions of buoyant density (BD) ranging from 1.620 g ml−1 to 1.642 g ml−1 and 1.584 g ml−1 to 1.608 g ml−1 were combined to constitute compiled ‘heavy
fractions’ and ‘light fractions’, respectively (Supplementary Figs. S1 and S2). Total community DNA was extracted from the original sediment core samples collected in July in 5-cm intervals
spanning 0–70 cm core depth. The populations of bacteria, archaea, and methanotrophs in the original sediments was estimated by qPCR targeting bacterial and archaeal 16S rRNA genes and
_pmoA_ with the primer sets Bac331F/Bac797R, Ar349F/Ar806R, and A189F/mb661R, respectively [34]. The primer sets Bac331F/ Bac797R, Ar349F/Ar806R have coverage of 68.4% and 71.6% of bacteria
and archaea in the SILVA rRNA database, respectively. Since all known methanotrophs have particulate MMO (pMMO) except for _Methylocella_ [35] and _Methyloferula_ [36], the gene _pmoA_
encoding pMMO has been widely used to study the taxonomic identity and community of methanotrophs in the environment [37]. The DNA from the original sediments were used as templates for
T-RFLP analysis as described previously [32, 38, 39]. The peak areas within each T-RFLP were converted to proportional peak areas and then were used for principal component analysis (PCA) to
screen for community structural patterns prior to more in-depth molecular analyses [38]. ANALYSES OF BACTERIAL AND ARCHAEAL 16S RRNA GENES PCR targeting bacterial and archaeal 16S rRNA
genes was performed on the following sample types: (1) total community DNA from the sediments homogenized from 25 to 50 cm deep (initial sediment used for incubations), (2) the heavy and
light fractions from the 13CH4-incubated samples unamended with inhibitors or electron acceptors, and (3) the heavy fractions from the 12CH4-incubated samples (unlabeled controls, unamended
with inhibitors or electron acceptors). The primer sets 27F/1392R and Ar309F/Ar915R were used to amplify bacterial and archaeal 16S rRNA genes. The PCR amplification and cloning were
performed in three replicates as described previously [32]. A total of 192 and 96 clones (averaging 64 and 32 clones from each replicate) were selected randomly from the heavy and light
fractions from the 13CH4-incubated samples, respectively. All 21 clones obtained from the heavy fractions of unlabeled controls were sequenced and analyzed using previously described methods
[32]. The high-quality sequences were dereplicated with the CAP3 sequence assembly program with 97% similarity [40]. The phylogenetic affiliations of bacterial sequences were classified
using the Ribosomal Database Project (RDP) (http://rdp.cme.msu.edu). Phylogenetic trees of archaeal clone sequences were constructed using MEGA X 10.1 software employing the
maximum-likelihood method based on Kimura 2-parameter model as described previously [32]. METAGENOMIC ANALYSIS Total DNA was extracted from the sediment cores (25–50 cm depth, homogenized)
and the heavy DNA fractions from the 13CH4-incubated samples (unamended with electron acceptors or inhibitors) for shotgun metagenomic analysis. Since the quantity of DNA in SIP fractions
was low, the heavy fractions from all the samples were merged into one sample. Genomic DNA was fragmented using sonication and subjected to shotgun metagenomic analysis by the Beijing
Genomics Institute (BGI, China). Paired-end fragment libraries with the insert size of 350 bp were constructed. Adapter-appended fragments were sequenced on HiSeq 2500 platform (Illumina).
Raw sequencing reads were filtered to obtain high-quality data (quality-filtered data) for subsequent analysis as described previously [41]. A percentage of 95.41–95.65% reads were filtered
and then assembled de novo with SOAP denovo2 [42] and Rabbit [43]. MetaGeneMark (version 2.10, default parameters) was used to predict open reading frames based on assembly results [44, 45].
Genes from different samples were combined together and clustered using CD-HIT (version 4.6.1) [46] to remove redundant sequences (sequence identity threshold 95% and alignment coverage
threshold 90%) [45]. The relative abundance of gene cluster representatives in each sample was calculated using the formula below as described previously [47]. $$G_i =
\frac{{\frac{{P_i}}{{L_i}}}}{{\mathop {\sum }\nolimits_{j = 1}^n \frac{{P_j}}{{L_j}}}}$$ Where _G__i_ is the relative abundance of gene _i_ in the sample, _P__i_ is the number of reads
mapped to gene _i_, _L__i_ is the length of gene _i_, and _n_ is the gene number in the assembled metagenomic data. The gene catalogs were aligned against the eggNOG database (2015-10), CAZy
database (2017-09), COG database (2014-11), Swiss-prot database (2017-07), the KEGG database (89.1), and CARD database (4.0) using DIAMOND with an e-value cutoff of 10−5 [45, 46] to search
the protein sequences. Ambiguously aligned sequencing reads were then reassigned to genes using Pathoscope v1.0, which uses a Bayesian framework to examine sequence of each read and mapping
quality within the context of a global reassignment [48]. Taxonomic assignment of the predicted genes was carried out using MEGAN (version 5.3) and subjected to BLASTX analysis using the
NCBI-nr database (2016-09) as described previously [49]. The relative abundance of members of each taxonomic level was approximated by calculating the number of the gene reads affiliated
with the taxonomic level to the total number of assembled reads per metagenome with an average assembly length of 13,697,259 bp and an average mapping rates of 31.05%. Clone sequences and
metagenomic data sets in this study have been submitted to the GenBank database and the NCBI Short Read Archive under accession numbers MN788533-MN788604 and SRP234857 (BioSample
SAMN13483815 and SAMN13483816 for the heavy DNA, and BioSample SAMN13483815 and SAMN13483816 for the total DNA of the original sediment), respectively. RESULTS BIOCHEMICAL CHARACTERIZATION
OF SEEP SEDIMENTS In May (ice-covered conditions), dissolved CH4 levels in porewater were similar along the core depth ranging from 136 to ~355 μM (Fig. 1). However, in July (ice-free),
porewater dissolved CH4 concentrations were low (<15 μM) in the upper sediments (0–10 cm), and, as the sediment depth increased, dissolved CH4 concentration increased and reached a
maximum of 1334 μM at a depth of 25 cm in the sediment, which was 4-fold higher than observed at the same depth in May. The content of HCl-extractable total Fe was 2526 mg kg−1 dry weight
(hereafter presented as mg kg−1) at the sediment-water interface, and increased to 14,846 mg kg−1 at the 10–15 cm depths, and then showed an overall decreasing trend with depth in May (Fig.
1D). The content of HCl-extractable Fe2+ showed a similar curve to the total Fe with higher values of 8326–14,698 mg kg−1 at the depths between 5 and 15 cm. The percent of HCl-extractable
Fe2+ to total Fe content was 77% at the sediment-water interface, increased to 95% at the depths between 10 and 15 cm, and averaged 94% for the remaining depths in May. The HCl-extractable
total Fe content was lower overall in the upper sediments in July than in May (Fig. 1E). The HCl-extractable total Fe content in the sediments in July showed an increasing trend from 10 to
60 cm with a drop at the depth of 45–50 cm in the sediments. In July, the percent of HCl-extractable Fe2+ to total Fe was 29% at the sediment-water interface, increased with depth to 67–68%
at the depth of 25–30 cm, and then kept stable with a range of 73–78% for the remaining depths. X-ray diffraction analysis showed that Fe3O4 and FeS2 were the main forms of Fe, accounting
for 1.65% and 0.16%, respectively, in the sediments in July, which was higher than the HCl-extractable Fe, likely owing to the low extraction of Fe3O4 (Supplementary Table S1). The bacterial
abundance was highest in the upper sediments (0–15 cm) in July with 2.3 × 108 to 5.2 × 108 copies g−1 (Fig. 2A). As the sediment depth increased from 20 to 70 cm, the bacterial abundance
gradually decreased from 6 × 106 to 1.3 × 106 copies g−1. Archaea and aerobic methanotrophs were detected along the entire 70-cm sediment core. The maximum abundance of archaea and aerobic
methanotrophs were detected at 10–15 cm with 2.7 × 106 and 3.7 × 105 copies g−1, respectively. The T-RFLP profiles of bacterial 16S rRNA genes from the original 0–70-cm sediment core depth
profile formed four groups in ordination space based on their depth ranges 0–10, 10–20, 20–55, and 55–70 cm (Fig. 2B and Supplementary Fig. S3). CH4 OXIDATION POTENTIAL IN ANOXIC SIP
MICROCOSMS Based on the principal component analysis of T-RFLP profiles and our finding that the position of the oxic-anoxic interface was located 15 cm below the sediment surface based on
the same core from the seep site [20], we selected the deeper 25–50 cm sediment depths as the subject of anoxic SIP experiments. During the SIP microcosm incubation, low CH4 consumption was
detected in the first 20 day (Fig. 3A, B). After 20 days, the CH4 consumption increased and varied over time. At the end of the experiment, the total CH4 consumption in the experimental SIP
microcosms was 0.028–0.030 mmol g wet weight−1. The CH4 oxidation potentials of the experimental sediments ranged from 0.126 to 0.136 μmol g−1 d−1 (Fig. 3C). The addition of EA, SM, and/or
BES did not have a significant effect on the CH4 oxidation potential in any of the treatments (_p_ > 0.587). We supplied 13CH4 and H218O to incubations in order to track the carbon and
oxygen sources into the headspace CO2. Within the 20-day incubation, the δ13C and δ18O values of the CO2 in the headspace of serum vials both increased with time (Fig. 3D), suggesting that
CH4-derived carbon and H2O-derived oxygen were converted into CO2. SIP TARGETING BACTERIAL 16S RRNA GENES We conducted bacterial 16S rRNA gene cloning and sequencing to identify bacteria
active in assimilating CH4-derived carbon. Three bacterial 16S rRNA gene clone libraries were established, including the heavy (BH) and light (BL) fractions from the 13CH4-incubated samples,
and the heavy fractions from the 12CH4-incubated samples as a control (BC). In the total, 135 bacterial 16S rRNA gene clones were sequenced from the BH library. Of these, 70.2% were
assigned to _Proteobacteria_. Within the _Proteobacteria_, 54.1% (73 out of 135 clones) were affiliated with _Methylobacter_, 7.4% were affiliated with _Methylotenera_, 3.0% belonged to the
genus _Rhodoferax_, and others were assigned to _Afipia_, unclassified _Acetobacteaceae_, unclassified _Alphaproteobacteria_, _Thiobacillus_, _Methylophilus_, and unclassified
_Betaproteobacteria_ (Fig. 4). The genera Gp6 and Gp7 in _Actinobacteria_ were dominant (accounting for 22.3% of the total clone sequences) in the BH library. _Bacteroidetes_,
_Actinobacteria_, _Verrucomicrobia_, _Planctomycetes_, and unclassified bacteria were also found in the BH library. Only a total of 14 bacterial 16S rRNA clones were obtained for the BC
library, possibly due to the low abundance of DNA in the heavy fraction from the control treatment. The genera _Rhodoferax_ and Gp6 detected in the BH library were also present in the BC
library. Of the 83 bacterial 16S rRNA clones sequenced from the BL library, 65.9% were assigned to _Proteobacteria_. The genera _Rhodoferax_ and _Methylobacter_ were both found in the BH and
BL libraries. SIP TARGETING ARCHAEAL 16S RRNA GENES We chose the primer set Ar309F/Ar915R to amplify archaeal 16S rRNA genes for sequencing. All 71 archaeal 16S rRNA gene sequences obtained
from the heavy fractions from the labeled DNA (CH) library belonged to the same operational taxonomic unit (OTU) (assigned by CAP3 sequence assembly program with 97% similarity), and had a
99% similarity to freshwater sediment clone Cad24-73 (AM851080). This clone was previously detected in sediments of Lake Cadagno and was affiliated with the AOM-associated archaeal (AAA)
clade of Euryarchaeota [50] (Fig. 5). Of the 58 sequences from the heavy fraction of the unlabeled DNA (CO) library, unclassified _Thermoprotei_ and unclassified _Methanosarcinales_ were
dominant and others were assigned to _Methanosaeta_, unclassified _Euryarchaeota_ and unclassified _Methanomicrobia_. Of the 85 archaeal 16S rRNA genes sequenced from the light fractions of
the labeled DNA (CL) library, 50.6% were assigned to unclassified _Methanosarcinales_, 22.4% belonged to unclassified _Thermoprotei_, 16.5% to _Methanobacterium_, and 8.2% to _Methanosaeta_.
TAXONOMIC PROFILING OF THE METAGENOMES We performed shotgun metagenomic sequencing for the heavy DNA from the 13CH4-incubated sediment and the total DNA from the original sediment. A
non-redundant catalog of 32,222 genes was constructed with an average 4.72 Gbp clean data. Taxonomic assignment showed that _Proteobacteria_, _Cyanobacteria_, _Bacteroidetes_,
_Acidobacteria_, _Actinobacteria_, and _Firmicutes_ predominated in the heavy (13C-labeled) DNA and the original sediment, accounting for 64.3–66.4% of the taxonomically assigned reads (Fig.
6). In the heavy DNA, _Proteobacteria_ was the dominant phylum, while _Cyanobacteria_ was the predominant microorganism in the original sediment. In the heavy DNA from the 13CH4-incubated
SIP sediment (Fig. 6C), sequences affiliated with genera containing known Gammaproteobacterial methanotrophs were detected, including _Methylobacter_, _Methylocaldum_, _Methylococcus_,
_Methylomarinum_, _Methylomicrobium_, _Methylomonas_, _Methylosarcina_, and _Methylovulum_, accounting for 46.0% of the taxonomically assigned reads. Among them, _Methylobacter_ (27.0%) was
the most abundant Gammaproteobacterial methanotroph. With the extended incubation time (240 days), CH4-derived carbon could have flowed into more microorganisms by crossing-feeding
processes, such as through formaldehyde secretion, CO2 emission, or decay of methanotrophic biomass followed by assimilation by other organisms [51]. Among the top 50 genera in assimilating
CH4-derived carbon, 24 genera were related with nitrogen, sulfide, metal (mainly iron), and electron transport such as _Desulfovibrio_, _Methylotenera_, _Pseudomonas_, _Geobacter,
Rhodoferax_, _Shewanella, Acidithiobacillus_, and _Chlorobium_ with the total relative abundance of 5.9% (Supplementary Table S2). _Acaryochloris_, a member of _Cyanobacteria_ was also among
the top 50 genera that assimilated CH4-derived carbon. _Cyanobacteria_ including _Pseudanabaena_, _Scytonema, Nostoc, Leptolyngbya, Oscillatoria_, and _Gloeocapsa_ were also listed the 100
genera assimilating CH4-derived carbon. “_Candidatus_ Metylomirabilis oxyfera” was not detected in the heavy DNA. In the original sediment microbial community, _Cyanobacteria_ was the most
abundant phylum. Of the top 50 genera, 42 were affiliated with the phylum _Cyanobacteria_, accounting for 54.6% of the taxonomically assigned reads (Fig. 6D). In the top 100 genera, 49
genera were non-Cyanobacterial with the relative abundance of 4.3% (Fig. 6E). Of them, 18 genera were detected in both the heavy DNA and the original sediment and accounted for 2.1% of the
assigned reads. Gammaproteobacterial methanotrophs including _Methylobacter, Methylomicrobium, Methylomonas_ and _Methylovulum_ and Alphaproteobacterial methanotroph _Methylosinus_ were
listed in the top 100 genera, accounting for 0.5% the taxonomically assigned reads. GENES INVOLVED IN CH4 OXIDATION, NITROGEN, HYDROGEN AND IRON METABOLISM The first step of CH4 oxidation to
methanol is catalyzed by MMO. The genes _pmoC, pmoA_, or _pmoB_ annotated as part of the pmo operon encoding pMMO (named _pmo_ genes) was detected in the original sediment and the heavy DNA
with a relative abundance of 0.0056% and 0.092%, respectively (Fig. 7). All _pmo_ genes were taxonomically assigned to cultured aerobic methanotrophs based on MEGAN. The gene _mmoX_,
encoding soluble MMO (sMMO), was not detected in the original sediment or the heavy DNA. Methanol is subsequently oxidized by either a calcium-dependent MxaF-type or a lanthanide-dependent
XoxF-type methanol dehydrogenase encoded by _mxa_ annotated as part of the mxa operon and _xoxF_, which were detected with the relative abundances of 0.135% and 0.019%, respectively, in the
heavy DNA. In addition to aerobic methanotrophs, some _mxa_ and _xoxF_ sequences were associated with methylotrophs. The _fdhA_ gene, encoding particulate cytochrome-linked formaldehyde
dehydrogenase, was detected in the original sediment and the heavy DNA, but it was not affiliated with aerobic methanotrophs. The relative abundance of genes involved in the
tetrahydromethanopterin (H4MPT)-dependent oxidation of formaldehyde to formate, including _fae, mtdB, mch_, and _ftr_ [52], ranged from 0.0001 to 0.002% in the original sediment and from
0.015 to 0.044% in the heavy DNA and were all associated with aerobic methanotrophs. The relative abundance of genes potentially involved in H4F-dependent oxidation of formaldehyde to
formate, including _mtdA_ and _fch_, ranged from 0.0004 to 0.002% in the original sediment and from 0.013 to 0.015% in the heavy DNA, however, _fhs_, encoding formyl-H4F synthetase, was not
detected. Additionally, the genes _gfa_, _frmA_, and _frmB_, potentially involved in glutathione-dependent formaldehyde oxidation, were not detected in the original sediment or the heavy
DNA, except for _frmA_, which was detected in the original sediment but was not associated with aerobic methanotrophs. These results suggest that formaldehyde was mainly oxidized by the
H4MPT-dependent pathway in the anoxic SIP incubation. Genes potentially involved in nitrogen metabolism, including nitrogen-fixation and denitrification, were also detected in the heavy DNA.
The reduction of nitrate to nitrite can be catalyzed by two different putative membrane-associated dissimilatory nitrate reductases, i.e., the cytoplasmic electrogenic enzyme complex NarGHI
(_narGHI_) (transmembrane nitrate reductase, NAR) and the periplasmic enzyme complex NapAB (_napAB)_ (periplasmic nitrate reductase, NAP) [26]. Of them, _nap_ was not detected in the
original sediment or the heavy DNA, but _narG_, _narH_, and _narI_ were present and were mainly associated with aerobic methanotrophs. The genes for _nasA_, encoding assimilatory nitrate
reductase was detected in the heavy DNA, but none were associated with aerobic methanotrophs. The gene annotated as encoding the copper-containing nitrite reductase, _nirK_, was detected in
the original sediment and the heavy DNA, of which 17.9% and 54.7% were associated with aerobic methanotrophs. However, we did not detect _nirS_, encoding the cytochrome cd1 nitrite reductase
in the original sediment or the heavy DNA. The relative abundance of _norBC_ genes, encoding nitric oxide reductase, ranged from 0.014 to 0.017% in the heavy DNA and most were associated
with aerobic methanotrophs. Additionally, the genes potentially involved in nitrogen fixation, including _nifD_, _nifH_, and _nifK_, and the gene _hao_ (annotated as encoding hydroxylamine
oxidoreductase), were also detected in the original sediment and the heavy DNA and were all associated with aerobic methanotrophs. The genes _hyaA_ and _hyaB_, encoding group 1d [NiFe]
hydrogenase, and the genes potentially involved in bidirectional hydrogenase including _hoxH, hoxY, hoxU_, and _hoxF_ were detected in the original sediment and were mainly associated with
aerobic methanotrophs and _Cyanobacteria_. Of them, 5.6–72.0% of _hoxH_, _hyaB_, and _hoxY_ were associated with aerobic methanotrophs. All _hyaA_, _hoxU_, and _hoxF_ genes detected were
affiliated with aerobic methanotrophs. The genes potentially involved in hydrogen metabolism including hydrogenase and bidirectional hydrogenase were also detected in the heavy DNA, of which
over 67.1% were associated with aerobic methanotrophs. Additionally, the homologs of genes _mtrA_ and _mtrC_, encoding mtrA and mtrC cytochrome, which may be potentially involved in iron
reduction [53], were detected in the heavy DNA. The homologs of gene _cycl_ were also detected in the original sediment and the heavy DNA that encodes Cyc1 protein, which is a member of the
cytochrome c4 family of high-redox-potential proteins and may be potentially involved in iron oxidation [54]. Over 72.2% of the detected homologs of gene _cyc1_ were affiliated with aerobic
methanotrophs. However, we did not detect _mtrB_ and _cyc2_ genes and their homologs, which are potentially involved in iron oxidation and reduction [52, 53], in the original sediment or the
heavy DNA. DISCUSSION In this study, we found that microorganisms typically classified as aerobic methanotrophs were present and active in assimilating CH4-derived carbon during anoxic
incubations using sediments collected from an active CH4 seep in an Arctic lake. Aerobic methanotrophs were present in sediments down to at least 70 cm deep at the active CH4 seep, despite
being under persistently anoxic conditions. In anoxic SIP mesocosms using lake sediments obtained from 25 to 50 cm deep, CH4 assimilation was dominated by Gammaproteobacterial methanotrophs,
including _Methylocaldum, Methylococcus, Methylomarinum, Methylomicrobium, Methylomonas_, _Methylosarcina_, _Methylovulum_, and especially _Methylobacter_. _Methylobacter_ has also been
reported to be abundant in the _Beggiatoa_ mats and the anoxic center of the Haakon Mosby Mud Volcano in CH4-rich sediments with an O2 penetration depth of only a few millimeters or less
[19, 55, 56]. Although counterintuitive, similar findings of aerobic methanotrophs thriving under anoxic conditions and actively oxidizing CH4 despite their obligate aerobe designation have
been reported from other environments as well [19, 22,23,24, 57, 58]. Metagenomic analyses of heavy DNA revealed that the Gammaproteobacterial methanotrophs active in our anoxic SIP sediment
experiment have substantial metabolic flexibility. In addition to having genes associated with the production of the CH4-oxidation intermediates such as methanol, formaldehyde, and formate
and the H4MPT-dependent oxidation of formaldehyde, methanotrophs that assimilated CH4-derived carbon had genes for nitrogen cycling and hydrogen processing. Despite the presence of genes
associated with nitrate reduction in methanotrophs, CH4 oxidation was unlikely to be coupled to denitrification in the SIP mesocosms or in situ, owing to the extremely low levels of
dissolved inorganic nitrogen (0.6–1.3 μM, including NH4+-N concentration of 0–0.7 μM and the NO3−-N concentration of 0.6 μM in July water samples). CH4 oxidation also was apparently not
linked to sulfate reduction, since amendment of mesocosms with sulfate or an inhibitor of sulfate reduction had no effect on CH4 oxidation. However, iron was notably abundant in sediments in
levels stoichiometrically sufficient to serve as an electron acceptor for oxidation of the high quantity of CH4 in the SIP mesocosms, where Fe3+ molar mass in sediments was 4.46 times of
that of CH4 consumption in the mesocosms (calculated by Fe3O4 based on the X-ray diffraction analysis, Supplementary Table S1; As noted previously, there is some possibility of iron
oxidation during sample storage that may have contributed to Fe3+ levels some degree) (Supplementary information). Organisms that derived carbon from CH4 included known iron reducers such as
_Desulfovibrio_ and _Shewanella_ [59]. Additionally, the genes possibly involved in iron oxidation and reduction such as _mtrAC_ and cyc1 were also present in the heavy 13C-DNA. Based on
the evidence, we propose that aerobic methanotrophs were active in assimilating CH4 or CH4-derived intermediates under anoxic conditions in these sediments, and that this might be coupled to
iron reduction, although further study is required to definitively determine which electron acceptor(s) are utilized in these sediments. Furthermore, these aerobic methanotrophs were
metabolically flexible, with the genetic potential to perform some nitrogen cycling and hydrogen metabolism processes that might aid in sustaining their anoxic methanotrophy under certain
conditions (Fig. 8). Aerobic methanotrophs are known to possess genes associated with nitrogen metabolism and can contribute to nitrogen cycling in the environment [26, 60, 61]. Although
denitrification was unlikely to be coupled to CH4 oxidation in our incubations or in situ due to low inorganic nitrogen levels, the active aerobic methanotrophs possessed the genetic
potential to do so, which might have relevance in more N-rich environments. Genes for denitrification, including _narGHI_, _nirK_, and _norBC_ (Fig. 8), have been proposed as a means of
energy conservation for aerobic methanotrophs under O2-limitated conditions [26]. In our study, _nap_ was not detected but _narG_, _narH_, and _narI_ were present in the original sediment
and the heavy DNA, and were mainly associated with aerobic methanotrophs, based on taxonomic classification by MEGAN. This indicated that aerobic methanotrophs could potentially utilize NAR
during denitrification rather than NAP, which provides theoretically greater energy conservation through pmf-driven ATP synthesis under anoxic conditions [26, 62]. More relevant to our
N-limited system, genes potentially involved in nitrogen fixation including _nifD_, _nifH_, and _nifK_, and the gene _hao_ annotated as encoding hydroxylamine oxidoreductase were also
detected in heavy DNA and were annotated as associated with aerobic methanotrophs. This indicated that some aerobic methanotrophs might potentially fix nitrogen and use hydroxylamine
oxidoreductase to oxidize and detoxify hydroxylamine [61], which might supply nitrogen for microbial metabolism. The aerobic methanotrophs active in our SIP mesocosms also possessed the
genetic potential for hydrogen metabolism. Molecular hydrogen is thought to be an alternative means for energy conservation in aerobic methanotrophs, providing an energetic advantage over
methanotrophy under O2-limited conditions [63]. Molecular hydrogen also can be used by aerobic methanotrophs such as _Methylococcus capsulatus_ (Bath) as a source of reducing power for CH4
oxidation, driving MMO activities in the presence or absence of O2 [64, 65]. However, the mechanism for hydrogen-driven MMO activity has not been investigated in detail. Respiratory-linked
_hyaAB_ hydrogenases affiliated with aerobic methanotrophs were present in our heavy DNA, and might potentially provide energy to sustain chemolithoautotrophic growth of aerobic
methanotrophs on hydrogen [66, 67]. Mixotrophic growth (oxidation of both hydrogen and CH4) in the thermoacidophile _Methylacidiphilum_ sp. RTK17.1 has been observed under O2-limited
conditions and was proposed to provide a competitive advantage over obligate methanotrophy at oxic/anoxic soil boundaries within geothermal environments [68]. Hydrogen metabolism in aerobic
methanotrophs has also been reported to increase the production of intracellular glycogen reservoirs under O2-limited conditions [63, 68], which can serve to store carbon and energy and to
maintain intracellular redox states. And, these hydrogen-driven MMO activities of aerobic methanotrophs have been observed under both O2-limited and anoxic conditions [64], making their role
in sediment systems worthy of future investigation. The diverse metabolic capabilities of the organisms assimilating CH4 in our mesocosms raised the question of which electron acceptor(s)
might be actively utilized by the consortium as it oxidized CH4 or its intermediates, such as methanol and formaldehyde (given the detection of H4MPT-dependent oxidation pathway, which is
common in AOM [9]). In addition to aerobic methanotrophs, microorganisms associated with the metabolism of nitrogen, sulfide, metal (mainly iron), and electron transport such as
_Desulfovibrio_, _Pseudomonas_, _Geobacter, Rhodoferax Shewanella, Acidithiobacillus_, and _Chlorobium_ were also active in assimilating CH4-derived carbon. As noted previously, conditions
in situ and in our incubations made denitrification and sulfate reduction highly unlikely, we suspected that the H4MPT-dependent oxidation of formaldehyde might be achieved mainly with metal
reduction, specifically iron, due to the sediments being very iron rich. ANME were also detected in the heavy DNA that had a 99% similarity to anaerobic freshwater sediment clone Cad24-73
(AM851080) [50], an organism taxonomically related to the _Methanoperedenaceae_ family, which has the ability to oxidize CH4 coupled to iron reduction [16, 17]. Moreover, two predicted genes
were taxonomically assigned to microbes that were abundant in the heavy DNA: _Acidithiobacillus_, the only autotrophic bacterial genus that includes species capable of anaerobic sulfur
oxidation with ferric iron as an electron acceptor [69, 70], and the iron-oxidizing sulfur bacterium _Chlorobium ferrooxidans_. The active cycling of iron might aid in providing electron
acceptors for the oxidation of CH4 and its metabolites. The potential free energy for CH4 oxidation coupled to iron reduction was energetically favorable in our SIP microcosms, based on
simplified free energy yield estimates: Fe(OH)3, CH4 + 8Fe(OH)3 + 15H+ → HCO3− + 8Fe2+ + 21H2O ∆G = −341.2 kJ mol−1 (Supplementary information). In other lake systems, the growth of
Gammaproteobacterial methanotrophs have been stimulated by the addition of Fe3+ under anoxic conditions with a CH4 assimilation of 7.5 ± 2.1 fmol C cell−1 d−1 [22]. And, in a sub-Arctic lake
sediment in Alaska, iron was also identified as a potential electron acceptor supporting CH4 oxidation [19]. The mechanism linking methanotrophs designated as aerobes with concurrent metal
reduction under anoxic conditions is still not clear. A co-culture-based SIP study of syntrophic AOM coupled to iron reduction revealed that methanotrophic bacterium _Methylomonas_ had
_mtd_, encoding methylene-H4MPT dehydrogenase, which was absent in the heavy DNA of syntrophic partner _Methanobacterium_. This was likely due to AOM intermediates being used as an electron
source for ferrihydrite reduction by the partner bacterium [71]. Additionally, potential homologs of the gene _mtrC_ (encoding mtrC cytochrome, which may be potentially involved in iron
reduction [53]) detected in the original sediment and the heavy DNA were affiliated with aerobic methanotrophs, although an more detailed analysis revealed them to be hypothetical membrane
fusion proteins and their function was not clear. This suggested that aerobic methanotrophs might potentially transfer electrons to solid minerals [72], although further verification is
needed. The use of solid external electron acceptors such as iron oxides and electrodes may be a survival strategy of methanotrophs to maintain their intracellular redox state in the
presence of electron donors such as formate and methanol [73]. Future experiments investigating the role and mechanisms of iron reduction by microbial consortia are warranted for iron-rich,
O2-limited systems such as our Arctic lake sediments. A lower ratio of HCl-extractable Fe2+ to total Fe in the deeper sediments in situ pointed to a high redox potential in the deeper seep
sediment under ice-free conditions compared to the ice-covered season, although we cannot entirely rule out that sample storage may have enabled iron oxidation leading to some measurement
error of Fe2+ (Fig. 1). The apparent iron redox gradient along the sediment depth profile might be attributed to extensive aeration of lake water and sediments during the open water season
due to wave action. The active turbation and settling of sediments at this seep might enable aerated water to penetrate deeper into sediments and oxidize iron. Additionally, the Fe and CH4
profiles in the CH4 seep site suggest that reduction of this oxidized iron (Fe3+) may be spatially correlated with the oxidation of CH4 or its intermediates like formaldehyde. Alternatively,
iron reduction may be linked with the decay of organic matter originating from microbial biomass, including the primary producers _Cyanobacteria_, which were abundant in sediment
communities. A high Fe content was detected in the sediment 10–15-cm deep under ice cover in May. This might be attributed to reduced Fe2+ being soluble and subject to upward flux to 10–15
cm, where it could be oxidized in the later summer/fall. Then, the Fe3+ might potentially serve as an electron acceptor reservoir supporting CH4 oxidation as ice cover occurred and O2 levels
declined. _Cyanobacteria_ were dominant members of our 25–50-cm-deep original sediment community, likely due to turbation and settling of sediments at this highly active seep (Fig. 6D).
Also, _Cyanobacteria_ assimilated CH4-derived carbon in anoxic SIP mesocosms, including members of the genera _Acaryochloris_, _Pseudanabaena_, _Scytonema, Nostoc, Leptolyngbya,
Oscillatoria_, and _Gloeocapsa_ (Fig. 6C). The presence of metabolically active _Cyanobacteria_ in anoxic mesocosms might be attributed to the cross-feeding of CH4-derived carbon, owing to
their ability to grow mixotrophically or heterotrophically on organic matter, which also might enable _Cyanobacteria_ to survive through non-photosynthetic mechanisms after burial deeper
into the sediment [74]. The analysis of δ18O enrichment of CO2 derived from 18O–H2O in the headspace of serum vials showed that it increased over time within the 20-day incubation (Fig. 3D).
There are several possible pathways for the conversion of 18O-H2O into 18O-CO2 such as (1) the degradation of organic matter, (2) hydrolysis of H2O into OH− and then conversion into CO2,
and (3) 18O exchange between CO2 and H2O [15, 75,76,77]. Further studies need to be conducted to determine which process(es) were involved in the conversion of 18O–H2O into 18O–CO2 in the
SIP mesocosms. Additionally, we also cannot rule out the possibility of some low-level 18O2 production from 18O–H2O via photosynthesis by _Cyanobacteria_ during the SIP incubation, followed
by the use of this labeled O2 in CH4 oxidation, since the serum vials were exposed to ambient laboratory lighting for 4–6 h every 20 days when determining gas concentrations and exchanging
headspace gas. Considering the minimal light exposure during the SIP experiment, we estimated that any possible O2 production from photosynthesis was very low (Supplementary information).
Although we also could not exclude rare O2 leakage during sampling or flushing of bottles, O2 produced in mesocosms on these rare occasions would be insufficient to explain the large
quantity of CH4 oxidized in the otherwise anoxic mesocosms. Taken together, our study indicated that aerobic methanotrophs were abundant and active in the deeper seep-associated sediments
and in the anoxic SIP mesocosms. Aerobic methanotrophs possessed metabolic flexibility to produce CH4 oxidation intermediates and to utilize them (i.e. via the H4MPT-dependent oxidation of
formaldehyde), as well as the potential capacity for hydrogen oxidation and denitrification using _narGHI_, _nirK_, and _norBC_. These capabilities might provide these organisms additional
means for survival through conserving energy and maintaining a favorable intracellular redox state under anoxic conditions. Based on the geochemistry of the lake and sediments, including low
inorganic nitrogen and sulfate levels, iron, which is highly abundant, was identified as the most likely potential electron acceptor. These findings contribute to the effort to understand
CH4 oxidation under anoxic conditions and to reconsider the role that methanotrophs designated as obligately aerobic may play in the CH4 cycle in the Arctic, especially lakes that experience
extended O2 limitations annually while ice covered. REFERENCES * Saunois M, Stavert AR, Poulter B, Bousquet P, Canadell JG, Jackson RB, et al. The global methane budget 2000-2017. Earth
Syst Sci Data. 2020;12:1561–623. Article Google Scholar * Reeburgh WS. Oceanic methane biogeochemistry. Chem Rev. 2007;107:486–513. Article CAS PubMed Google Scholar * Weber T, Wiseman
NA, Kock A. Global ocean methane emissions dominated by shallow coastal waters. Nat Commun. 2019;10:4584. Article PubMed PubMed Central CAS Google Scholar * He R, Wooller MJ, Pohlman
JW, Quensen J, Tiedje JM, Leigh MB. Shifts in identity and activity of methanotrophs in arctic lake sediments in response to temperature changes. Appl Environ Microb. 2012;78:4715–23.
Article CAS Google Scholar * Phelps AR, Peterson KM, Jeffries MO. Methane efflux from high-latitude lakes during spring ice melt. J Geophys Res Atmos. 1998;103:29029–36. Article CAS
Google Scholar * Walter KM, Smith LC, Chapin FS. Methane bubbling from northern lakes: present and future contributions to the global methane budget. Philos Trans A Math Phys Eng Sci.
2007;365:1657–76. CAS PubMed Google Scholar * Wik M, Varner RK, Anthony KW, MacIntyre S, Bastviken D. Climate-sensitive northern lakes and ponds are critical components of methane
release. Nat Geosci. 2016;9:99–105. Article CAS Google Scholar * Knittel K, Boetius A. Anaerobic oxidation of methane: progress with an unknown process. Annu Rev Microbiol.
2009;63:311–34. Article CAS PubMed Google Scholar * Timmers PHA, Welte CU, Koehorst JJ, Plugge CM, Jetten MSM, Stams AJM. Reverse methanogenesis and respiration in methanotrophic
archaea. Archaea. 2017;2017:1654237. Article PubMed PubMed Central CAS Google Scholar * Shen LD, Ouyang L, Zhu Y, Trimmer M. Active pathways of anaerobic methane oxidation across
contrasting riverbeds. ISME J. 2019;13:752–66. Article CAS PubMed Google Scholar * Valenzuela EI, Cervantes FJ. The role of humic substances in mitigating greenhouse gases emissions:
Current knowledge and research gaps. Sci Total Environ. 2021;750:141677. Article CAS PubMed Google Scholar * Orphan VJ, House CH, Hinrichs KU, McKeegan KD, DeLong EF. Multiple archaeal
groups mediate methane oxidation in anoxic cold seep sediments. Proc Natl Acad Sci USA. 2002;99:7663–8. Article CAS PubMed PubMed Central Google Scholar * Orphan VJ, House CH, Hinrichs
KU, McKeegan KD, DeLong EF. Methane-consuming archaea revealed by directly coupled isotopic and phylogenetic analysis. Science. 2001;293:484–7. Article CAS PubMed Google Scholar * Haroon
MF, Hu S, Shi Y, Imelfort M, Keller J, Hugenholtz P, et al. Anaerobic oxidation of methane coupled to nitrate reduction in a novel archaeal lineage. Nature. 2013;500:567–70. Article CAS
PubMed Google Scholar * Cai C, Leu AO, Xie GJ, Guo JH, Feng YX, Zhao JX, et al. A methanotrophic archaeon couples anaerobic oxidation of methane to Fe(III) reduction. ISME J.
2018;12:1929–39. Article CAS PubMed PubMed Central Google Scholar * Ettwig KF, Zhu B, Speth D, Keltjens JT, Jetten MSM, Kartal B. Archaea catalyze iron-dependent anaerobic oxidation of
methane. Proc Natl Acad Sci USA. 2016;113:12792–6. Article CAS PubMed PubMed Central Google Scholar * Leu AO, Cai C, Mcllroy SJ, Southam G, Orphan VJ, Yuan ZG, et al. Anaerobic methane
oxidation coupled to manganese reduction by members of the Methanoperedenaceae. ISME J. 2020;14:1030–41. Article CAS PubMed PubMed Central Google Scholar * Ettwig KF, Butler MK, Le
Paslier D, Pelletier E, Mangenot S, Kuypers MMM, et al. Nitrite-driven anaerobic methane oxidation by oxygenic bacteria. Nature. 2010;464:543–8. Article CAS PubMed Google Scholar *
Martinez-Cruz K, Leewis MC, Herriott IC, Sepulveda-Jauregui A, Anthony KW, Thalasso F, et al. Anaerobic oxidation of methane by aerobic methanotrophs in sub-Arctic lake sediments. Sci Total
Environ. 2017;607:23–31. Article PubMed CAS Google Scholar * He R, Wooller MJ, Pohlman JW, Quensen J, Tiedje JM, Leigh MB. Diversity of active aerobic methanotrophs along depth profiles
of arctic and subarctic lake water column and sediments. ISME J. 2012;6:1937–48. Article CAS PubMed PubMed Central Google Scholar * Bowman JP, Sly LI, Nichols PD, Hayward AC. Revised
taxonomy of the methanotrophs: description of Methylobacter gen. nov., validation of Methylosinus and Methylocystis species, and a proposal that the family Methylococcaceae includes only the
group I methanotrophs. Int J Syst Bacteriol. 1993;43:735–53. Article Google Scholar * Oswald K, Milucka J, Brand A, Hach P, Littmann S, Wehrli B, et al. Aerobic gammaproteobacterial
methanotrophs mitigate methane emissions from oxic and anoxic lake waters. Limnol Oceanogr. 2016;61:S101–18. Article Google Scholar * Cabrol L, Thalasso F, Gandois L, Sepulveda-Jauregui A,
Martinez-Cruz K, Teisserenc R, et al. Anaerobic oxidation of methane and associated microbiome in anoxic water of Northwestern Siberian lakes. Sci Total Environ. 2020;736:139588. Article
CAS PubMed Google Scholar * Milucka J, Kirf M, Lu L, Krupke A, Lam P, Littmann S, et al. Methane oxidation coupled to oxygenic photosynthesis in anoxic waters. ISME J. 2015;9:1991–2002.
Article CAS PubMed PubMed Central Google Scholar * Kalyuzhnaya MG, Yang S, Rozova ON, Smalley NE, Clubb J, Lamb A, et al. Highly efficient methane biocatalysis revealed in a
methanotrophic bacterium. Nat Commun. 2013;4:2785. Article CAS PubMed Google Scholar * Kits KD, Klotz MG, Stein LY. Methane oxidation coupled to nitrate reduction under hypoxia by the
Gammaproteobacterium Methylomonas denitrificans, sp. nov. type strain FJG1. Environ Microbiol. 2015;17:3219–32. Article CAS PubMed Google Scholar * Eberl DD. Quantitative mineralogy of
the Yukon River system: variations with reach and season, and determining sediment provenance. Am Mineral. 2004;89:1784–94. Article CAS Google Scholar * Lipson DA, Raab TK, Goria D,
Zlamal J. The contribution of Fe(III) and humic acid reduction to ecosystem respiration in drained thaw lake basins of the Arctic Coastal Plain. Glob Biogeochem Cycles 2013;27:399–409.
Article CAS Google Scholar * Li WB, Yao J, Tao PP, Guo MT, Feng XY, He YN, et al. A comparative study on two extraction procedures in speciation of iron in municipal solid waste. J Hazard
Mater. 2010;182:640–8. Article CAS PubMed Google Scholar * Oremland RS, Taylor BF. Sulfate reduction and methanogenesis in marine sediments. Geochim Cosmochim Acta. 1978;42:209–14.
Article CAS Google Scholar * Glassburn CL, Potter BA, Clark JL, Reuther JD, Bruning DL, Wooller MJ. Strontium and oxygen isotope profiles of sequentially sampled modern bison (bison bison
bison) teeth from interior Alaska as proxies of seasonal mobility. Arctic. 2018;71:183–200. Article Google Scholar * He R, Wooller MJ, Pohlman JW, Catranis C, Quensen J, Tiedje JM, et al.
Identification of functionally active aerobic methanotrophs in sediments from an arctic lake using stable isotope probing. Environ Microbiol. 2012;14:1403–19. Article CAS PubMed Google
Scholar * Leigh MB, Pellizari VH, Uhlik O, Sutka R, Rodrigues J, Ostrom NE, et al. Biphenyl-utilizing bacteria and their functional genes in a pine root zone contaminated with
polychlorinated biphenyls (PCBs). ISME J. 2007;1:134–48. Article CAS PubMed Google Scholar * Swan BK, Ehrhardt CJ, Reifel KM, Moreno LI, Valentine DL. Archaeal and bacterial communities
respond differently to environmental gradients in anoxic sediments of a California hypersaline lake, the salton sea. Appl Environ Microb. 2010;76:757–68. Article CAS Google Scholar *
Dedysh SN, Liesack W, Khmelenina VN, Suzina NE, Trotsenko YA, Semrau JD, et al. Methylocella palustris gen. nov., sp. nov., a new methane-oxidizing acidophilic bacterium from peat bogs,
representing a novel subtype of serine-pathway methanotrophs. Int J Syst Evol Microbiol. 2000;50:955–69. Article CAS PubMed Google Scholar * Vorobev AV, Baani M, Doronina NV, Brady AL,
Liesack W, Dunfield PF, et al. Methyloferula stellata gen. nov. sp. nov. an acidophilic, obligately methanotrophic bacterium that possesses only a soluble methane monooxygenase. Int J Syst
Evol Microbiol. 2011;61:2456–63. Article CAS PubMed Google Scholar * Kolb S, Knief C, Stubner S, Conrad R. Quantitative detection of methanotrophs in soil by novel pmoA-targeted
real-time PCR assays. Appl Environ Microbiol. 2003;69:2423–9. Article CAS PubMed PubMed Central Google Scholar * Fierer N, Schimel JP, Holden PA. Influence of drying–rewetting frequency
on soil bacterial community structure. Micro Ecol. 2003;45:63–71. Article CAS Google Scholar * Fierer N, Jackson RB. The diversity and biogeography of soil bacterial communities. Proc
Natl Acad Sci USA. 2006;103:626–31. Article CAS PubMed PubMed Central Google Scholar * Huang XQ, Madan A. CAP3: a DNA sequence assembly program. Genome Res. 1999;9:868–77. Article CAS
PubMed PubMed Central Google Scholar * Ma RC, Chu YX, Wang J, Wang C, Leigh MB, Chen Y, et al. Stable-isotopic and metagenomic analyses reveal metabolic and microbial link of aerobic
methane oxidation coupled to denitrification at different O2 levels. Sci Total Environ. 2020;764:142901. Article PubMed CAS Google Scholar * Luo RB, Liu BH, Xie YL, Li ZY, Huang WH, Yuan
JY, et al. SOAPdenovo2: an empirically improved memory-efficient short-read de novo assembler. GigaScience. 2012;1:18. Article PubMed PubMed Central Google Scholar * You MS, Yue Z, He
WY, Yang XH, Yang G, Xie M, et al. A heterozygous moth genome provides insights into herbivory and detoxification. Nat Genet. 2013;45:220–5. Article CAS PubMed Google Scholar * Zhu WH,
Lomsadze A, Borodovsky M. Ab initio gene identification in metagenomic sequences. Nucleic Acids Res. 2010;38:e132. Article PubMed PubMed Central CAS Google Scholar * Guo JH, Peng YZ,
Fan L, Zhang L, Ni BJ, Kartal B, et al. Metagenomic analysis of anammox communities in three different microbial aggregates. Environ Microbiol. 2016;18:2979–93. Article CAS PubMed Google
Scholar * Li WZ, Godzik A. Cd-hit: a fast program for clustering and comparing large sets of protein or nucleotide sequences. Bioinformatics. 2006;22:1658–9. Article CAS PubMed Google
Scholar * Liu SP, Chen QL, Zou HJ, Yu YJ, Zhou ZL, Mao J, et al. A metagenomic analysis of the relationship between microorganisms and flavor development in Shaoxing mechanized huangjiu
fermentation mashes. Int J Food Microbiol. 2019;303:9–18. Article CAS PubMed Google Scholar * Francis OE, Bendall M, Manimaran S, Hong CJ, Clement NL, Castro-Nallar E, et al. Pathoscope:
Species identification and strain attribution with unassembled sequencing data. Genome Res. 2013;23:1721–9. Article CAS PubMed PubMed Central Google Scholar * He Y, Feng XY, Fang J,
Zhang Y, Xiao X. Metagenome and metatranscriptome revealed a highly active and intensive sulfur cycle in an oil-immersed hydrothermal chimney in Guaymas basin. Front Microbiol. 2015;6:1236.
Article PubMed PubMed Central Google Scholar * Schubert CJ, Vazquez F, Losekann-Behrens T, Knittel K, Tonolla M, Boetius A. Evidence for anaerobic oxidation of methane in sediments of a
freshwater system (Lago di Cadagno). FEMS Microbiol Ecol. 2011;76:26–38. Article CAS PubMed Google Scholar * He R, Wooller MJ, Pohlman JW, Tiedje JM, Leigh MB. Methane-derived carbon
flow through microbial communities in arctic lake sediments. Environ Microbiol. 2015;17:3233–50. Article CAS PubMed Google Scholar * Vorholt JA. Cofactor-dependent formaldehyde oxidation
in methylotrophic bacteria. Arch Microbiol. 2002;178:239–49. Article CAS PubMed Google Scholar * Garber AI, Nealson KH, Okamoto A, McAllister SM, Chan CS, Barco RA, et al. FeGenie: a
comprehensive tool for the identification of iron genes and iron gene neighborhoods in genome and metagenome assemblies. Front Microbiol. 2020;11:37. Article PubMed PubMed Central Google
Scholar * Barco A, Emerson D, Sylvan JB, Orcutt BN, Meyers MEJ, Ramírez GA, et al. New insight into microbial iron oxidation as revealed by the proteomic profile of an obligate
iron-oxidizing chemolithoautotroph roman. Appl Environ Microbiol. 2015;81:5927–37. Article CAS PubMed PubMed Central Google Scholar * De Beer D, Sauter E, Niemann H, Kaul N, Foucher JP,
Witte U, et al. In situ fluxes and zonation of microbial activity in surface sediments of the Hakon Mosby Mud Volcano. Limnol Oceanogr. 2006;51:1315–31. Article Google Scholar * Lösekann
T, Knittel K, Nadalig T, Fuchs B, Niemann H, Boetius A, et al. Diversity and abundance of aerobic and anaerobic methane oxidizers at the Haakon Mosby mud volcano, Barents Sea. Appl Environ
Microb. 2007;73:3348–62. Article CAS Google Scholar * Blees J, Niemann H, Wenk CB, Zopfi J, Schubert CJ, Kirf MK, et al. Micro-aerobic bacterial methane oxidation in the chemocline and
anoxic water column of deep south-Alpine Lake Lugano (Switzerland). Limnol Oceanogr. 2014;59:311–24. Article CAS Google Scholar * Reid T, Chaganti SR, Droppo IG, Weisener CG. Novel
insights into freshwater hydrocarbon-rich sediments using metatranscriptomics: opening the black box. Water Res. 2018;136:1–11. Article CAS PubMed Google Scholar * Weber KA, Achenbach
LA, Coates JD. Microorganisms pumping iron: anaerobic microbial iron oxidation and reduction. Nat Rev Microbiol. 2006;4:752–64. Article CAS PubMed Google Scholar * Ward N, Larsen Ø,
Sakwa J, Bruseth L, Khouri H, Durkin AS, et al. Genomic insights into methanotrophy: the complete genome sequence of Methylococcus capsulatus (Bath). PLoS Biol. 2004;2:1616–28. Article CAS
Google Scholar * Versantvoort W, Pol A, Jetten MSM, van Niftrik L, Reimann J, Kartal B, et al. Multiheme hydroxylamine oxidoreductases produce NO during ammonia oxidation in
methanotrophs. Prot Natl Acad Sci USA. 2020;117:24459–63. Article CAS Google Scholar * Richardson DJ, Berks BC, Russell DA, Spiro S, Taylor CJ. Functional, biochemical and genetic
diversity of prokaryotic nitrate reductases. Cell Mol Life Sci. 2001;58:165–78. Article CAS PubMed Google Scholar * Carere CR, McDonald B, Peach HA, Greening C, Gapes DJ, Collet C, et
al. Hydrogen oxidation influences glycogen accumulation in a verrucomicrobial methanotroph. Front Microbiol. 2019;10:1873. Article PubMed PubMed Central Google Scholar * Hanczár T, Csáki
R, Bodrossy L, Murrell JC, Kovács KL. Detection and localization of two hydrogenases in Methylococcus capsulatus (Bath) and their potential role in methane metabolism. Arch Microbiol.
2002;177:167–72. Article PubMed CAS Google Scholar * Shah NN, Hanna ML, Jackson KJ, Taylor RT. Batch cultivation of Methylosinus trichosporium OB3b. 4: production of hydrogen-driven
soluble or particulate methane monooxygenase activity. Biotechnol Bioeng. 1995;45:229–38. Article CAS PubMed Google Scholar * Mohammadi S, Pol A, van Alen TA, Jetten MSM, Op den Camp
HJM. Methylacidiphilum fumariolicum SolV, a thermoacidophilic ‘Knallgas’ methanotroph with both an oxygen-sensitive and -insensitive hydrogenase. ISME J. 2017;11:945–58. Article CAS PubMed
Google Scholar * Carere CR, Hards K, Houghton KM, Power JF, McDonald B, Collet C, et al. Mixotrophy drives niche expansion of Verrucomicrobial methanotrophs. ISME J. 2017;11:2599–610.
Article PubMed PubMed Central Google Scholar * Orata FD, Meier-Kolthoff JP, Sauvageau D, Stein LY. Phylogenomic analysis of the Gammaproteobacterial methanotrophs (Order Methylococcales)
calls for the reclassification of members at the genus and species levels. Front Microbiol. 2018;9:3162. Article PubMed PubMed Central Google Scholar * Kucera J, Sedo O, Potesil D,
Janiczek O, Zdrahal Z, Mandl M. Comparative proteomic analysis of sulfur-oxidizing Acidithiobacillus ferrooxidans CCM 4253 cultures having lost the ability to couple anaerobic elemental
sulfur oxidation with ferric iron reduction. Res Microbiol. 2016;167:587–94. Article CAS PubMed Google Scholar * Kucera J, Zeman J, Mandl M, Cerna H. Stoichiometry of bacterial anaerobic
oxidation of elemental sulfur by ferric iron. Antonie van Leeuwenhoek. 2012;101:919–22. Article PubMed Google Scholar * He QX, Yu LP, Li JB, He D, Cai XX, Zhou SG. Electron shuttles
enhance anaerobic oxidation of methane coupled to iron (III) reduction. Sci Total Environ. 2019;688:664–72. Article CAS PubMed Google Scholar * Jing XX, Wu YC, Shi L, Peacock CL, Ashry
NM, Gao CH, et al. Outer membrane c-type cytochromes OmcA and MtrC play distinct roles in enhancing the attachment of Shewanella oneidensis MR-1 cells to goethite. Appl Environ Microbiol.
2020;86:e01941–20. Article CAS PubMed PubMed Central Google Scholar * Tanaka K, Vokoe S, Igarashi K, Takashino M, Ishikawa M, Hori K, et al. Extracellular electron transfer via outer
membrane cytochromes in a methanotrophic bacterium Methylococcus capsulatus (Bath). Front Microbiol. 2018;9:2905. Article PubMed PubMed Central Google Scholar * Kamalanathan M, Dao LHT,
Chaisutyakorna P, Gleadow R, Beardall J. Photosynthetic physiology of Scenedesmus sp (Chlorophyceae) under photoautotrophic and molasses-based heterotrophic and mixotrophic conditions.
Phycologia. 2017;56:666–74. Article CAS Google Scholar * Qu LR, Wang C, Bai E. Evaluation of the 18O-H2O incubation method for measurement of soil microbial carbon use efficiency. Soil
Biol Biochem. 2020;145:107802. Article CAS Google Scholar * Kapiluto Y, Yakir D, Tans P, Berkowitz B. Experimental and numerical studies of the 18O exchange between CO2 and water in the
atmosphere–soil invasion flux. Geochim Cosmochim Acta. 2007;71:2657–71. Article CAS Google Scholar * Zeebe RE. Kinetic fractionation of carbon and oxygen isotopes during hydration of
carbon dioxide. Geochim Cosmochim Acta. 2014;139:540–52. Article CAS Google Scholar Download references ACKNOWLEDGEMENTS This work was conducted under BLM permit (FF095556), and North
Slope Borough permits (NSB 09-0478 and NSB 10-018). Any use of trade, firm, or product names is for descriptive purposes only and does not imply endorsement by the U.S. Government. This work
was supported by funding from United States Department of Energy National Energy Technology Laboratory (Grant DE-NT000565) and National Natural Science Foundation of China with Grants No.
91851109 and 41671245 and Natural Science Foundation of Zhejiang province with Grant No. LZ20E080002. Support for the UAF molecular instrumentation in the Institute of Arctic Biology
Genomics Core Laboratory was provided by an Institutional Development Award (IDeA) from the National Institute of General Medical Sciences of the National Institutes of Health under grant
number 2P20GM103395. The findings and conclusions in this article are those of the authors and do not necessarily reflect the official views of the NIH. AUTHOR INFORMATION AUTHORS AND
AFFILIATIONS * Zhejiang Provincial Key Laboratory of Solid Waste Treatment and Recycling, School of Environmental Science and Engineering, Zhejiang Gongshang University, Hangzhou, China Ruo
He * Department of Environmental Engineering, Zhejiang University, Hangzhou, China Ruo He, Jing Wang & Yi-Xuan Chu * Institute of Arctic Biology, University of Alaska Fairbanks,
Fairbanks, AK, USA Ruo He & Mary Beth Leigh * U.S. Geological Survey, Woods Hole Coastal and Marine Science Center, Woods Hole, MA, USA John W. Pohlman * State Key Laboratory of Soil and
Sustainable Agriculture, Institute of Soil Science, Chinese Academy of Sciences, Nanjing, China Zhongjun Jia * Alaska Stable Isotope Facility, Water and Environmental Research Center,
University of Alaska Fairbanks, Fairbanks, AK, USA Matthew J. Wooller * College of Fisheries and Ocean Sciences, University of Alaska Fairbanks, Fairbanks, AK, USA Matthew J. Wooller Authors
* Ruo He View author publications You can also search for this author inPubMed Google Scholar * Jing Wang View author publications You can also search for this author inPubMed Google
Scholar * John W. Pohlman View author publications You can also search for this author inPubMed Google Scholar * Zhongjun Jia View author publications You can also search for this author
inPubMed Google Scholar * Yi-Xuan Chu View author publications You can also search for this author inPubMed Google Scholar * Matthew J. Wooller View author publications You can also search
for this author inPubMed Google Scholar * Mary Beth Leigh View author publications You can also search for this author inPubMed Google Scholar CORRESPONDING AUTHORS Correspondence to Ruo He
or Mary Beth Leigh. ETHICS DECLARATIONS COMPETING INTERESTS The authors declare no competing interests. ADDITIONAL INFORMATION PUBLISHER’S NOTE Springer Nature remains neutral with regard to
jurisdictional claims in published maps and institutional affiliations. SUPPLEMENTARY INFORMATION SUPPLEMENTARY INFORMATION RIGHTS AND PERMISSIONS Reprints and permissions ABOUT THIS
ARTICLE CITE THIS ARTICLE He, R., Wang, J., Pohlman, J.W. _et al._ Metabolic flexibility of aerobic methanotrophs under anoxic conditions in Arctic lake sediments. _ISME J_ 16, 78–90 (2022).
https://doi.org/10.1038/s41396-021-01049-y Download citation * Received: 08 October 2020 * Revised: 19 June 2021 * Accepted: 23 June 2021 * Published: 09 July 2021 * Issue Date: January
2022 * DOI: https://doi.org/10.1038/s41396-021-01049-y SHARE THIS ARTICLE Anyone you share the following link with will be able to read this content: Get shareable link Sorry, a shareable
link is not currently available for this article. Copy to clipboard Provided by the Springer Nature SharedIt content-sharing initiative