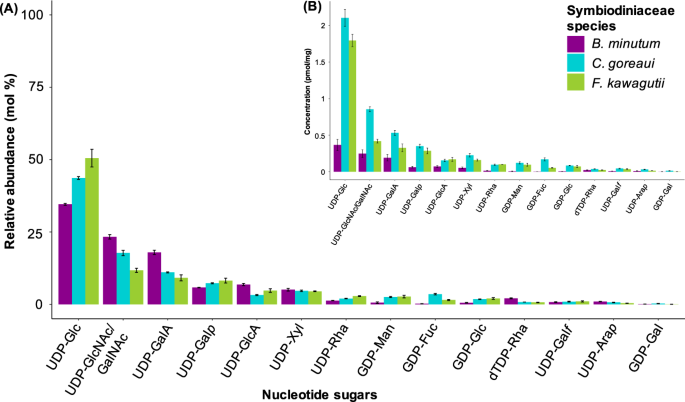
Cell surface carbohydrates of symbiotic dinoflagellates and their role in the establishment of cnidarian–dinoflagellate symbiosis
- Select a language for the TTS:
- UK English Female
- UK English Male
- US English Female
- US English Male
- Australian Female
- Australian Male
- Language selected: (auto detect) - EN
Play all audios:

ABSTRACT Symbiodiniaceae algae are often photosymbionts of reef-building corals. The establishment of their symbiosis resembles a microbial infection where eukaryotic pattern recognition
receptors (e.g. lectins) are thought to recognize a specific range of taxon-specific microbial-associated molecular patterns (e.g. glycans). The present study used the sea anemone,
_Exaiptasia diaphana_ and three species of Symbiodiniaceae (the homologous _Breviolum minutum_, the heterologous-compatible _Cladocopium goreaui_ and the heterologous-incompatible _Fugacium
kawagutii_) to compare the surface glycomes of three symbionts and explore the role of glycan–lectin interactions in host–symbiont recognition and establishment of symbiosis. We identified
the nucleotide sugars of the algal cells, then examined glycans on the cell wall of the three symbiont species with monosaccharide analysis, lectin array technology and fluorescence
microscopy of the algal cell decorated with fluorescently tagged lectins. Armed with this inventory of possible glycan moieties, we then assayed the ability of the three Symbiodiniaceae to
colonize aposymbiotic _E. diaphana_ after modifying the surface of one of the two partners. The Symbiodiniaceae cell-surface glycome varies among algal species. Trypsin treatment of the alga
changed the rate of _B. minutum_ and _C. goreaui_ uptake, suggesting that a protein-based moiety is an essential part of compatible symbiont recognition. Our data strongly support the
importance of D-galactose (in particular β-D-galactose) residues in the establishment of the cnidarian–dinoflagellate symbiosis, and we propose a potential involvement of L-fucose, D-xylose
and D-galacturonic acid in the early steps of this mutualism. SIMILAR CONTENT BEING VIEWED BY OTHERS DINOFLAGELLATE SYMBIONTS ESCAPE VOMOCYTOSIS BY HOST CELL IMMUNE SUPPRESSION Article 29
April 2021 CORAL HOLOBIONT CUES PRIME _ENDOZOICOMONAS_ FOR A SYMBIOTIC LIFESTYLE Article Open access 20 April 2022 INTRACELLULAR BACTERIA ARE COMMON AND TAXONOMICALLY DIVERSE IN CULTURED AND
_IN HOSPITE_ ALGAL ENDOSYMBIONTS OF CORAL REEFS Article Open access 08 February 2021 INTRODUCTION Symbiodiniaceae are single-celled alveolate protists of the phylum Dinoflagellata.
Dinoflagellates inhabit temperate and tropical areas as phototrophs, heterotrophs, parasites and symbionts, but the Symbiodiniaceae are most widely recognized as intracellular photosymbionts
of reef-building corals [1]. Coral–Symbiodiniaceae symbiosis is a mutualism where the symbiont benefits from inorganic nutrients coming from host metabolism, and the coral host receives a
large proportion of photosynthate produced by the dinoflagellates, thus providing the coral partner with most of its energy requirements and therefore underpinning the entire coral reef
ecosystem [2]. The family Symbiodiniaceae comprises at least nine genera [1, 3]. The physiological differences among the genera—and even within genera—are enormous [4], and dramatically
influence the physiology of the coral holobiont (i.e. the coral and its associated microbiota) and its ability to cope with environmental stressors [5]. Indeed, it is pivotal for the coral
host to engage in symbiosis with specific symbiont types to survive [6]. The majority of coral species have a horizontal transmission mode and must acquire suitable symbionts from the
surrounding environment [7, 8]. Symbiosis establishment via horizontal mechanisms involves attraction, recognition and uptake of ‘the right’ symbionts [2]. Although there is some knowledge
about the processes of attraction [9, 10] and uptake [11, 12, 13], little is known on the mechanisms of symbiotic specificity and host–symbiont selection. Inter-partner signalling molecules
involved in symbiosis establishment between corals and symbiotic dinoflagellates are still being explored [14]. The initiation of this symbiosis has been compared to the recognition of a
pathogen during microbial infection [15]. In this model, the molecular crosstalk between the two symbiotic partners is mediated by the specific interaction between taxon-specific
microbial-associated molecular patterns (MAMPs) and pattern recognition receptors (PRRs). A widespread MAMP-PRR system is the glycan–lectin interaction that acts as a lock-and-key mechanism
in both beneficial and detrimental symbioses [16], and this is proposed as a model for establishment of the cnidarian–dinoflagellate mutualism [15]. The cell surface of symbiotic
dinoflagellates is populated with glycoconjugates, with some glycan motifs similar among species and others unique to each species [17]. Two types of sugar linkages, mannose-mannose and
galactose-β(1-4)-N-acetylglucosamine, have been reported in the algal cell walls [18]. Conversely, mannose-binding lectin (Millectin; [19]), two N-acetyl-D-galactosamine binding proteins
(Tachylectin-2-like lectin AtTL-2; [20]) and two D-galactose binding lectins, SLL-2 [21] and CecL [22], have been isolated from several coral species and shown to be involved in various
phases of symbiosis establishment. The well-described N-glycan biosynthesis pathway in yeast, plants and humans [23] is also present in Symbiodiniaceae and is a key factor in
cnidarian–dinoflagellate symbiosis establishment, highlighting the importance of the symbiont’s cell-surface sugar composition in this process. The majority of the N-glycan types found on
the symbiont surface were mannose-rich, further supporting the role of mannose in symbiosis onset [24]. The sugars N-acetyl-D-galactosamine [20, 25], fucose [24] and α-glucose [25, 26] were
also found on the symbiont cell surface. Several studies thus support the involvement of glycan–lectin interactions in the establishment of symbiosis between cnidarians and Symbiodiniaceae,
but their roles require further exploration. Here, we adopted a model organism approach to explore the molecules at the interface of the cnidarian–dinoflagellate symbiosis and investigate
the hypothesis that a glycan–lectin interaction plays a role in the initiation of this relationship. The model is the sea anemone, _Exaiptasia diaphana_, which forms symbioses with members
of the Symbiodiniaceae [15]. Importantly, _E. diaphana_ can be freed of all symbionts by chemical bleaching, and then inoculated with new symbionts, thus providing an ideal platform to
dissect host/symbiont recognition at a molecular level. We previously developed a matrix of host–symbiont compatibilities [27] with homologous-compatible (_Breviolum minutum_),
heterologous-compatible (_Cladocopium goreaui_) and incompatible (_Fugacium kawagutii_) symbionts and three different anemone genotypes [28]. We hypothesized that the compatible symbionts
(_B. minutum_ and _C. goreaui_) have similar cell-surface structures that fit the lock-and-key mechanism of the host and are different from the ones on the cell surface of incompatible algae
(_F. kawagutii_). Based on this concept, we explored and compared the composition of the three Symbiodiniaceae cell surfaces. A multi-step approach, comprising both well-established [20,
29, 30] and innovative methods [31], was adopted to profile the cell surface of these Symbiodiniaceae. In this way, we created a list of potential molecular candidates, that we then
strategically altered to investigate their role(s) during the first stages of symbiosis. MATERIALS AND METHODS EXPERIMENTAL ORGANISMS Three anemone genotypes (AIMS2, AIMS3 and AIMS4; [28])
of the cnidarian model _E. diaphana_ sourced from the Great Barrier Reef (GBR) were used in this study. Polyps were kept in reconstituted seawater (RSS, [27]) in a growth chamber (LE-509,
Thermoline Scientific) under constant temperature (27 °C), 12:12-h light:dark photoperiod cycle and 15-μmol photons m−2 s−1 irradiance (white + red LED lights, EDOLED), and fed with freshly
hatched _Artemia_ sp. nauplii ad libitum twice per week. Three Symbiodiniaceae species were used in this work: _B. minutum_ (MMSF 01, ITS2 type B1), _C. goreaui_ (SCF 055-01.10, ITS2 type
C1) and _F. kawagutii_ (SCF 089.01, ITS2 type F1). These algae were chosen based on their different compatibilities with the anemone host [27]. The _B. minutum_ culture was isolated from the
_E. diaphana_ anemones mentioned above [27], while _C. goreaui_ and _F. kawagutii_ were obtained from the Australian Institute of Marine Science (AIMS) where they were originally isolated
from the GBR-sourced corals _Acrocopora tenuis_ and _Pocillopora damicornis_, respectively. All cultures were maintained in 1-L Schott bottles with 0.2-μm membrane vented caps in a growth
chamber (740FHC LED, HiPoint) under constant temperature (27 °C), 12:12-h light:dark photoperiod cycle, and 60-μmol photons m−2 s−1 of light. NUCLEOTIDE SUGAR AND MONOSACCHARIDE COMPONENT
ANALYSIS OF SYMBIODINIACEAE CELL WALLS The nucleotide sugar and cell-wall monosaccharide compositions of _B. minutum_, _C. goreaui_ and _F. kawagutii_ were profiled using methods well
established in higher plants [31, 32, 33]. For the nucleotide sugar analysis, Symbiodiniaceae cultures were harvested after 5 h of exposure to light. Fifty milligrams of algae per sample
were resuspended in ice-cold methanol/chloroform (1:1 mixed solution), transferred into custom lysing matrix D 2-ml tubes (MP Biomedicals), ground in liquid nitrogen using a cryomill, then
vortexed and placed at −20 °C for 2 h. Following the addition of 400-µL ice-cold water, the samples were centrifuged at 20,000 × _g_ at 4 °C and the upper phase was transferred into 15-ml
tubes placed on ice. The addition of ice-cold water and centrifugation steps were repeated two more times, and the supernatants were combined with the previously collected aqueous phase.
Samples were frozen in liquid nitrogen and freeze-dried overnight. Sample purification was undertaken by solid phase extraction, then nucleotides sugars were detected and quantified by
liquid chromatography tandem mass spectrometry (LC-MS/MS) using a 4,000 QTRAP LC/MS/MS system (SCIEX) equipped with a TurboIonSpray source (SCIEX) and an Agilent 1,100 Series Capillary LC
System. The following nucleotide sugar standards were used in this study: UDP–α-D-xylose, UDP–β-L-arabinopyranose and UDP–α-D-galacturonic acid (Carbosource Services, Complex Carbohydrate
Research Center); UDP–α-D-glucuronic acid, UDP–α-D-glucose, UDP–α-D-galactose, UDP–N-acetyl-α-D-glucosamine, UDP–N-acetyl-α-D-galactosamine, GDP–α-D-mannose, GDP–β-L-fucose, GDP-Glc
(Sigma-Aldrich) and UDP–β-L-arabinofuranose (Peptides International; [32]). Data were acquired using Analyst 1.5.1 (SCIEX) and nucleotide sugars were quantified using MultiQuant 2.1 software
(SCIEX) by linear regression of the peak areas [33]. To analyse the algal cell-wall monosaccharide composition, we took 300 mg of pelleted algae, and ground the cells in liquid nitrogen as
described above. To obtain cell-wall alcohol insoluble residues (AIR), the homogenate was incubated in 100% EtOH at 100 °C for 30 min under constant shaking at 1,400 rpm. The AIR was then
pelleted by centrifugation at 20,000 × _g_ for 5 min. The pellet was washed twice in 70% EtOH and subsequently in acetone, and then left to dry overnight. Single sugar units
(monosaccharides) were released by hydrolysis of AIRs with 2-N trifluoroacetic acid (TFA) and incubation at 120 °C for 1 h. The TFA solution was removed by evaporation overnight in a vacuum
concentrator. The monosaccharide analysis was performed using High Performance Anion Exchange Chromatography coupled with Pulsed Amperometric Detection (HPAEC-PAD). Three replicates for each
Symbiodiniaceae species were diluted and subsequently analysed on an ICS 6,000 (Dionex Corporation, Sunnyvale, CA) essentially as described in Rautengarten et al. 2014 [32], with the
exception that the first isocratic elution step was performed with 4 mM to separate xylose and mannose, and while in a separate run 8 mM was used to separate N-acetyl-galactosamine and
rhamnose. Standards comprised L-fucose, L-rhamnose, L-arabinose, C-galactose, D-galactose, D-xylose, D-galacturonic acid, D-glucuronic acid, N-acetylgalactosamine and N-acetylglucosamine.
Both N-acetylgalactosamine and N-acetylglucosamine were TFA-treated prior to the run. A run of a standard mixture was performed with each sample set to enable sample quantitation by linear
regression. A one-way Anova (or a non-parametric Kruskal–Wallis test if normality criteria were not met) was performed to compare the relative amount of all monosaccharides found within a
Symbiodiniaceae species and of each individual monosaccharide among _B. minutum_, _C. goreaui_ and _F. kawagutii_. Pairwise comparisons were performed post hoc with Tukey’s tests or
non-parametric Dunn test (_p_ > 0.05). CONFOCAL MICROSCOPY OF SYMBIODINIACEAE CELL SURFACE Fluorescent molecular probes conjugated with eight different lectins were used to explore their
affinity for the glycoconjugates populating the cell surface of _B. minutum_, _C. goreaui_ and _F. kawagutii_. Symbiodiniaceae cultures were divided into four aliquots of 1.5 × 106 cells for
each species and treatment. The pelleted cells were resuspended in 4% paraformaldehyde (PFA)/1× PBS, fixed for 24 h at 4 °C, washed in 1× PBS and stored at 4 °C. Three days later, each
sample was washed (4,000 × _g_) three times in 1× PBS, then one of the fluorescent lectins was added to the algal solution at a final concentration of 1 mg/ml [29]. The fluorescent lectin
conjugates used in this study, and their glycan affinities, are described in Table 1. After a 1-h incubation at room temperature in the dark, samples were washed three times in 1× PBS and
cells resuspended in a final volume of 100 μl. Five microliters of each sample were placed in four wells of a teflon printed microscope slide (ProSciTech), and then mounted with ProLong gold
antifade mountant (ThermoFisher scientific). Unstained controls were also prepared to profile the innate fluorescence of all algal strains. Samples were visualised with a Nikon A1R confocal
laser scanning microscope with the NIS-Element software. The acquisition of signals from specific fluorophores was obtained by recording variable emission bandwidth with the virtual band
mode. A 409-nm laser was used to detect chlorophyll autofluorescence from dinoflagellate cells, while the fluorophores Texas Red and AlexaFluor 568, 594 and 488 were specifically excited by
the lasers reported in Table 1. An oil immersion objective of ×60 magnification and 1.4 numerical aperture was used to image three different sections of each well, hence 12 observations were
made for each algal species and lectin treatment. The image of the algal species and lectin was unmixed against its negative control to remove any background signal caused by algal
chlorophyll autofluorescence. A macro was developed to process Nd2 files using Fiji™ and obtain the mean fluorescence intensity (MFI) of the signal provided by the lectin binding to the
symbiont surface. Twenty-five algal cells were randomly selected from each image, and hence 300 cells were analysed for each sample of a Symbiodiniaceae species incubated with each lectin.
Data were tested for normality with the Shapiro–Wilk test and, when parametric assumptions were not met, we used the Kruskal–Wallis test to explore the fluorescence intensity profiles of
different lectins binding to the surface of a Symbiodiniaceae species. Finally, the Tukey’s test was used for multiple comparisons (_p_ > 0.05). LECTIN ARRAY OF SYMBIODINIACEAE
CELL-SURFACE GLYCOPROTEINS To isolate glycoproteins from the Symbiodiniaceae cell surface, we used guanidinium chloride (GuHCl) extraction [34]. We confirmed that the extraction of
cell-surface glycoproteins/proteins did not result in lysis of the algal cell by using Calcofluor-white to stain the cellulosic algal cell wall [35]. Calcofluor-stained algae were visualised
with a Nikon A1R confocal laser scanning microscope with a 405-nm laser to detect stained cellulose and a 409-nm laser to detect chlorophyll autofluorescence from dinoflagellate cells. For
each algal species, an aliquot of 1 × 108 cells/ml was centrifuged at 1500 × _g_, washed twice in 1x PBS, resuspended in 3 ml of 6-M GuHCl and divided into two tubes. After 4 h of incubation
at RT under constant shaking at 800 rpm, cells were pelleted at 15,000 × _g_ for 30 min at RT and discarded; the supernatant of the two tubes was then concentrated and combined with an
Amicon Ultra MWCO 3-KDa filter, and the protein concentrations of the samples were checked with the Pierce BCA Protein Assay. Each algal species had _n_ = 4 replicates. Samples were run on a
Ray Biotech Lectin array 95, which has 95 different lectins (Supplementary information 1). One milligram per millilitre of glycoprotein suspension was run on the lectin array by Crux
Biolabs Australia. The binding of glycoproteins to different lectins produced fluorescence intensity signals informative of a certain glycan abundance. The data were background-corrected,
tested for normality and a one-way Anova used to explore the binding of a certain lectin among Symbiodiniaceae cell-surface glycoproteins. Finally, the Tukey’s test was used for multiple
comparisons (_p_ > 0.05), and the variation in _B. minutum_, _C. goreaui_ and _F. kawagutii_ cell-surface glycomes was visualised using principal component analysis (PCA). CELL-SURFACE
ALTERATION AND HOST–SYMBIONT INOCULATION EXPERIMENTS A total of 1053 anemones (oral disc diameter of ∼5 mm) was chemically bleached of algal symbionts as previously described [36]. After
bleaching, anemones were kept in RSS and fed with brine shrimp. One week prior to inoculation with Symbiodiniaceae symbionts, feeding was discontinued, and anemones were transferred to
12-well plates such that each 12-well plate contained _n_ = 3 anemone genotype replicates, organized randomly to minimize well effect. Each 12-well plate had _n_ = 3 technical replicates,
all altered with the same enzymatic or masking treatment and inoculated with one symbiont type. The anemone surface lectins were masked with the sugars D-glucose, D-mannose, D-galactose,
methyl-α- and β-D-galactose, L-fucose, D-xylose, D-galacturonic acid, D-glucuronic acid and L-rhamnose, all at a concentration of 10 mM (Table 2; [20]). Organisms were incubated in RSS
supplemented with one of the sugars for an hour under the conditions described above, and subsequently inoculated with one of the three Symbiodiniaceae species. Control organisms did not
receive any sugar prior to symbiont inoculation. The symbiont cell surface was altered via digestion with one of the two enzymes: α-amylase [29] or trypsin [18, 26, 29, 30]. Enzyme
specificities are reported in Table 2. Algal cells were sampled in the exponential growth phase, pelleted and resuspended in a digestion solution composed of RSS and one of the enzymes used
in the study. After a 2-h digestion step at 27 °C in the dark in a shaking incubator at 90 rpm, the symbionts were washed twice in RSS and used immediately to inoculate the bleached,
unmodified anemones. Control organisms received untreated symbionts. For both host and symbiont surface modification experiments, inoculations were performed as previously described [27].
Given that most Symbiodiniaceae cell-surface glycans recover within 48–72 h post-enzymatic cleaving [30], anemones were anesthetized with MgCl2 2 days post-inoculation, and tentacles then
sampled, fixed and microscopically analysed to assess symbiont cell densities in _E. diaphana_ [27]. Data were log-transformed to achieve normality and a generalized linear model (GLM) with
a Gaussian distribution was used to test the influence of _E. diaphana_ genotype (i.e. AIMS2, 3 and 4), Symbiodiniaceae species (i.e. _B. minutum_, _C. goreaui_ and _F. kawagutii_) and
treatment (i.e. enzymatic treatment of symbiont surface or masking of host lectins with carbohydrates) on symbiont uptake by the host (as number of symbiont cells/mm2 in anemone tentacles).
Analyses were conducted in R v. 3.6.1 [37] with the package Tidyverse [38]. The GLM specification for the model was: $$ {{{\boldsymbol{glm}}}}({{{\boldsymbol{N}}}} \sim
{{{{{\bf{host}}}}}}\;{{{{{\bf{genotype}}}}}} \ast {{{{{\bf{symbiont}}}}}}\;{{{{{\bf{species}}}}}} \ast {{{{{\bf{treatment}}}}}},\;{{{{{\bf{family}}}}}} = \\ {{{{{\bf{gaussian}}}}}}\left(
{{{{{{\bf{link}}}}}} = {{{\mathrm{log}}}}} \right))$$ Best model selection was performed by comparing the full model with all the effects and interactions against the model without each of
the effects or interactions and confirmed using the Akaike Information Criterion (AIC, 15395.97). Analysis of variance was used to test the significance of the overall fixed effects fitted
in the model. RESULTS NUCLEOTIDE SUGAR AND MONOSACCHARIDE COMPOSITION ANALYSIS OF SYMBIODINIACEAE CELL WALLS The surface of the symbiont cell constitutes an intricate mesh of cellulose,
glycoproteins and polysaccharides [17]. In eukaryotes, matrix polysaccharides are synthesized from activated sugar substrates called nucleotide sugars, most of which are transferred by
nucleotide sugar transporters from the cytosol into the Golgi apparatus where they are assembled into glycan polymers by various glycosyltransferases, and then transported via vesicles to
the cell wall [31]. Therefore, we decided to investigate the nucleotide sugar profiles from whole cells to gain insight into possible end products present in the dinoflagellate wall. We
detected various nucleotide sugars in the three species of Symbiodiniaceae investigated (Fig. 1). The respective sugar residues can be present in cell-wall polymers, glycoproteins and/or
surface glycans. We then examined the monosaccharide composition of insoluble cell-wall extracts from each of the three Symbiodiniaceae species (Fig. 2). All cell-wall monosaccharides
identified were also evident in their respective precursor form in the nucleotide sugar analysis (Fig. 1). The most abundant monosaccharide detected in the three species was glucose (Fig.
2). D-mannose was the only monosaccharide for which the relative amount did not statistically differ among the three Symbiodiniaceae species (_p_ > 0.05; Fig. 2). The relative amounts of
L-fucose, L-rhamnose, D-galactose, D-glucose, D-xylose and D-galacturonic acid differed among species (_p_ < 0.05; Fig. 2), while D-glucuronic acid was dissimilar between _B. minutum_ and
_F. kawagutii_ only (_p_ < 0.05; Fig. 2). L-arabinose, N-acetyl-galactosamine and -glucosamine were present in trace amounts. We therefore did not further investigate the effect of these
monosaccharides on symbiosis establishment. CONFOCAL MICROSCOPY OF SYMBIODINIACEAE CELL SURFACES The MFI profile of different lectin conjugates varied among the three Symbiodiniaceae
species (Fig. 3). The lectins ConA, GS-II, LPA, PhaL, SBA, UEA and WGA bound significantly to the surface of _B. minutum_. The _C. goreaui_ surface was populated by glycans with an affinity
to ConA, GS-II, LPA, PhaL, PNA, SBA and UEA. The surface of _F. kawagutii_ exhibited significant binding for all the lectin conjugates used in the study. ConA, which specifically binds to
terminal D-mannose and D-glucose residues, had a stronger intensity profile in _C. goreaui_ compared with _B. minutum_ and _F. kawagutii_ (_p_ < 0.001). The latter two species showed
similar binding of the probe to their cell surfaces. The lectins GS-II, LPA, PhaL, SBA and UEA all bound to _B. minutum_, _C. goreaui_ and _F. kawagutii_, and showed different MIFs among
symbiont species. _C. goreaui_ and _F. kawagutii_ presented different amounts of D-galactose on their cell surfaces, as revealed with the lectin probe PNA (_p_ < 0.001); however, the
lectin WGA identified a dissimilar abundance of glycoconjugates containing N-acetyl-D-glucosamine residues on _B. minutum_ and _F. kawagutii_ (_p_ < 0.001). LECTIN ARRAY OF
SYMBIODINIACEAE CELL-SURFACE GLYCOPROTEINS Confocal microscopy showed an intact Calcofluor-stained cell wall surrounding the Symbiodiniaceae cells after the GuHCl treatment (Supplementary
information 2), which we interpret as the extraction procedure not disrupting the majority of cells. Fifty-four percent of the lectins showed a difference in their affinity to the three
Symbiodiniaceae species (Supplementary information 1 and 3). In line with the confocal microscopy results, the Symbiodiniaceae surface glycome varied among _B. minutum_, _C. goreaui_ and _F.
kawagutii_, and PCA showed that cell-surface glycoproteins clustered according to symbiont species (Fig. 4). The presence/absence of D-mannose, D-glucose, D-galactose, L-fucose,
N-acetyl-D-galactosamine and N-acetyl-D-glucosamine identified via confocal microscopy was confirmed by the lectin array, but the array did not detect any statistically supported difference
among algal species for the lectins PhaL, SBA, UEA and WGA (Supplementary information 1 and 3). This discrepancy may be attributed to the sensitivity of each method, as we discuss later in
this work. Lectins of the array that were specific for NANA showed a light binding to the glycoproteins of the three algal cells (Supplementary information 3), as also shown by the binding
of the LPA lectin probe (Fig. 3). Sialic acids, usually characteristics of higher invertebrates, have been found composing a small percentage of the Symbiodiniaceae N-glycome [24]. Future
directions should explore the role of these molecules in the algal cell. CELL-SURFACE ALTERATION AND HOST–SYMBIONT INOCULATION EXPERIMENTS The density of _B. minutum_ and _C. goreaui_
populating _E. diaphana_ at 48 hpi varied considerably (Fig. 5 and Table 3). Congruent with findings in our previous study [27], _E. diaphana_ genotype influenced the start of symbiosis
(GLM, df = 2, _p_ < 0.001; Supplementary information 4), although a clear pattern between host identity and symbiosis establishment was not found. Symbiont species (GLM, df = 1, _p_ <
0.001), treatment (GLM, df = 12, _p_ < 0.001) and the interaction of the three factors (GLM, df = 24, _p_ < 0.001) significantly affected the establishment of new associations. The
homologous species (_B. minutum_) colonised the anemones rapidly, whereas the compatible heterologous species (_C. goreaui_) also colonised the animals, but at a lower density, and the
incompatible strain (_F. kawagutii_) did not measurably colonise the hosts (Fig. 5 and Table 3). After exposing aposymbiotic anemones to certain monosaccharides, we observed significantly
lower in hospite _B. minutum_ and _C. goreaui_ cell densities at 48 hpi for D-galactose (_p__B_ < 0.001; _p__C_ < 0.05), L-fucose and D-xylose (_p__B_ < 0.001; _p__C_ < 0.05).
Incubation of the aposymbiotic hosts with D-glucose, D-mannose or D-galacturonic acid resulted in lower algal densities (compared to the controls) for _B. minutum,_ but revealed no
difference for _C. goreaui_ (_p__B_ < 0.001; _p__C_ > 0.05). Exposing the hosts to α-D-galactose and L-rhamnose had no effect on symbiosis establishment with any of the two compatible
symbionts (_p_ > 0.05), whereas the presence of β-D-galactose resulted in a super-infection for both _B. minutum_ and _C. goreaui_ (_p_ < 0.001). The inability of _F. kawagutii_ to
colonise _E. diaphana_ did not change (_p_ > 0.05) as a result of any of the monosaccharide host-masking trials (Fig. 5 and Table 3). Incubation of _E. diaphana_ with D-glucuronic acid
was lethal to the anemones, hence it was not possible to collect data for this treatment. Digesting proteins at the symbiont surface with the enzyme trypsin prior to inoculation
significantly affected the uptake of both compatible symbionts (_p__B_ < 0.001; _p__C_ < 0.05), severely reducing the success of _B. minutum_ but marginally increasing _C. goreaui_
cell density (Fig. 5 and Table 3). Alpha-amylase had no influence on the density of either _B. minutum_ or _C. goreaui_ found in the host compared to the control (_p_ > 0.05; Fig. 5 and
Table 3). The infectivity of _F. kawagutii_ for all genotypes of _E. diaphana_ did not differ significantly (_p_ > 0.05) from the control after α-amylase or trypsin pre-digestion (Fig. 5
and Table 3). DISCUSSION We explored the involvement of glycan–lectin interactions in the establishment of endosymbiosis between the sea anemone, _E. diaphana_ and three species of
Symbiodiniaceae algae, the homologous _B. minutum_, the heterologous-compatible _C. goreaui_ and the heterologous-incompatible _F. kawagutii_. As expected, we found good concordance between
the nucleotide sugar repertoire in the three Symbiodiniaceae species and our monosaccharide analysis, which is consistent with these precursors finding their way into glycan structures such
as those making up the cell walls. The cell-surface glycome varies among _B. minutum_, _C. goreaui_ and _F. kawagutii_, as shown by the monosaccharide analysis (Fig. 2), confocal microscopy
(Fig. 3) and the lectin array results (Fig. 4 and Supplementary information 3). GLUCOSE AND MANNOSE D-glucose stands out as highly abundant in both the nucleotide sugar pools and in the
cell-wall extracts of the three Symbiodiniaceae species (Figs. 1 and 2). The symbiont cell wall is predominantly composed of cellulose [17], a polysaccharide consisting of β-linked
D-glucose, so the abundance of this molecule across the three algal species is not surprising. Binding of the lectin molecular probe ConA, which is specific for D-glucose (and D-mannose), to
all the three algal species (Fig. 3) is also consistent with cellulose in the algal cell walls. _B. minutum_, _C. goreaui_ and _F. kawagutii_ cell-surface glycoproteins bound to several
lectins specific for glucose and mannose in the lectin array study (i.e. LcHA and PSA; Supplementary information 1 and 3). When we treated the anemones with D-glucose prior to inoculation
with symbionts, it significantly decreased the colonisation success of the homologous _B. minutum_ but had no impact on the colonisation success of _C. goreaui_ and _F. kawagutii_ (Fig. 5
and Table 3). D-mannose was the only sugar that did not differ significantly in mole percentage amongst the three species of Symbiodiniaceae (Fig. 2). Previous work has shown that
high-mannose glycans constitute 52% of the _B. minutum_ cell-surface N-glycome [24], and the most abundant protein on the cell surface of symbiotic dinoflagellates found by Lin and
co-workers bears a terminal mannose residue [18]. Here, lectins specific for high mannose such as BC2L-A, CALSEPA, GRFT and ORYSATA recognised glycoproteins of the three Symbiodiniaceae
species in the array experiment (Supplementary information 1 and 3). Furthermore, two mannose-binding lectins, ‘Millectin’ from _Acropora millepora_ [19] and ‘PdC lectin’ from _Pocillopora
damicornis_ [39], bind around the algae in the gastrodermis of the host [39] and are characterised by extensive sequence variation of the binding region, which may reflect the capacity to
recognise both beneficial symbionts and pathogens [19]. Here, treating the anemones with D-mannose prior to inoculation with symbionts decreased the colonisation success of the homologous
_B. minutum_ but had no effect on experiments with _C. goreaui_ and _F. kawagutii_ (Fig. 5 and Table 3). ConA negatively influenced symbiosis establishment between the coral _Fungia
scutaria_ and its symbionts [26], but, in the case of _A. tenuis_, the lectin had no effect on symbiotic colonisation [29]. Taken together, these results suggest that both D-mannose and
D-glucose residues are likely important functional components of the symbiont surface that participate in recognition of Symbiodiniaceae and maintenance of the mutualism, rather than just
being crucial monosaccharides responsible for the discrimination between compatible and incompatible symbionts. D-GALACTURONIC ACID The relative amount of D-galacturonic acid was different
among Symbiodiniaceae species, being more abundant in the compatibles _B. minutum_ and _C. goreaui_ compared to the non-compatible _F. kawagutii_ (Fig. 2). When we incubated the anemones in
D-galacturonic acid, colonisation success remained unaltered for _F. kawagutii_ and decreased for both _B. minutum_ and _C. goreaui_, although the decrease was only statistically supported
for _B. minutum_ (Fig. 5 and Table 3). We speculate that D-galacturonic acid may be a component of the glycoproteins involved in the recognition of the compatible symbionts. D-GALACTOSE The
presence of D-galactose molecules on the symbiont cell wall (Fig. 2) was confirmed via confocal microscopy with the lectin probe PNA (Fig. 3), and with the binding of algal surface
glycoproteins to galactose-specific lectins on the array, such as MNAG, RCA120 and RCA60 (Supplementary information 1 and 3). Interestingly, monosaccharide analysis showed D-galactose to be
more abundant in or on the cell wall of the homologous _B. minutum_ compared to the heterologous _C. goreaui_ and the incompatible _F. kawagutii_ (Fig. 2). Previous studies reported the
presence of D-galactose on the surface of several Symbiodiniaceae species [24, 25], and the colonisation of _A. tenuis_ larvae during symbiosis establishment was negatively influenced by the
presence of the sugar [20]. Here, D-galactose significantly decreased colonisation success of the homologous _B. minutum_ and the heterologous-compatible _C. goreaui_ in _E. diaphana_,
compared to the control (Fig. 5 and Table 3). Thus, several lines of evidence support the involvement of D-galactose in the establishment of symbiosis between cnidarians and Symbiodiniaceae.
For instance, the D-galactose-binding lectin SLL-2 has been isolated from the coral _Sinularia lochmodes_. This protein was localised surrounding the symbiont cells when resident in the
gastrodermis of the host, and in vitro experiments showed the ability of SLL-2 to arrest the dinoflagellate in the typical symbiotic state, viz non-dividing and non-motile [21, 40, 41, 42].
Another lectin (CeCL) that binds to carbohydrate chains with a D-galactosyl moiety was found in _Fungia echinate_, and—similar to SLL-2—CeCL transformed Symbiodiniaceae cells from a
flagellated and motile state to a coccoid and non-motile form [22]. Considering that the activity of CeCL on the symbiont cells was concentration-dependent [22], and that in our study
D-galactose was found to be more abundant in the compatible symbionts than the incompatible one, this raises the question whether the relative amount of a certain glycan has an impact on
symbiont selection. Another open question is whether the stereochemistry of a sugar is important in symbiont selection. To further explore the possible role of D-galactose in symbiont
selection, we utilised different conformations of the molecule, namely methyl-α- or methyl-β-D-galactose, which are fixed isomers due to the methyl group. Intriguingly, the methyl-α-sugar
had no effect on host colonisation, whereas methyl-β-D-galactose significantly altered the establishment of symbiosis between the host and compatible symbionts, by reducing the number of _B.
minutum_ but increasing the number of _C. goreaui_ present in the host at 48 hpi (Fig. 5 and Table 3). We conclude that D-galactose, in particular D-galactose when it is β-linked rather
than α-linked, is a crucial player of the lock-and-key mechanism responsible for recognising suitable symbionts during the establishment of symbiosis. FUCOSE AND XYLOSE Like D-galactose,
L-fucose and D-xylose are more abundant in extracts from the homologous _B. minutum_ than the heterologous _C. goreaui_ and _F. kawagutii_ (Fig. 2), and the lectin probe UEA confirmed the
presence of L-fucose exposed on the surface of symbiont cells (Fig. 3). Accordingly, PA-IIL and RSFUC, specific for fucose, bound to the surface glycome of the three algal species in the
lectin array (Supplementary information 1 and 3). Both carbohydrates have been associated with Symbiodiniaceae before, as N-glycan glycosyltransferases for fucose (fucosyltransferases) and
xylose (xylosyltransferases) are encoded in the algal genome [24]. Here, we show that both L-fucose and D-xylose decrease the colonisation success of _B. minutum_ and _C. goreaui_ in _E.
diaphana_ (Fig. 5 and Table 3). Although the role of these sugar residues at the initiation of symbiosis needs further exploration, these results suggest a function in the recognition of
compatible algal types. N-ACETYL HEXOSAMINE SUGARS Our HPAEC-PAD analysis detected only small traces of N-acetylglucosamine and N-acetylgalactosamine among the cell-wall monosaccharides of
_B. minutum_, _C. goreaui_ and _F. kawagutii_ (<1.5 mol%). Nevertheless, cell-surface glycoproteins of the three algal species bound several N-acetyl groups-specific lectins (i.e. GS-II,
PhaL, SBA and WGA), either in the fluorescence microscopy assay or lectin array or both. Although Logan and co-workers showed identical binding of these probes to various Symbiodiniaceae
species [25], our confocal microscopy study found that they bind differently to the surface of our strains of _B. minutum_, _C. goreaui_ and _F. kawagutii_ from the GBR (Fig. 3). In
particular, the incompatible _F. kawagutii_ presented high levels of N-acetyl-D-glucosamine compared to the compatibles _B. minutum_ and _C. goreaui_ (Fig. 3). In a previous study, two
N-acetyl-D-galactosamine binding lectins were isolated from the scleractinian _A. tenuis_, and seem to be involved in the acquisition of Symbiodiniaceae of the _Symbiodinium_ and
_Durusdinium_ genera [20]. In another study, carbohydrates with N-acetylglucosamine residues decreased the acquisition of compatible symbionts in _A. tenuis_. The
N-acetyl-D-glucosamine-binding lectin ActL attracted compatible Symbiodiniaceae to the coral host, suggesting a role in chemotactic attraction prior to recognition and uptake of the symbiont
to start symbiosis [43]. Perhaps, N-acetyl residues characterize symbionts incompatible with our host, _E. diaphana_ from the GBR. The role of N-acetylated sugars remains to be determined,
and further experiments are needed to uncover the role of these molecules in the cnidarians–Symbiodiniaceae symbiosis. LARGE AND Α-LINKED POLYSACCHARIDES, AND L-RHAMNOSE Treating the three
symbiont species with α-amylase or challenging them against _E. diaphana_ in the presence of L-rhamnose, did not alter the ability of the symbionts to colonize _E. diaphana_ (Fig. 5 and
Table 3). Starch-like structures are not typical components of dinoflagellate cell walls, so it is not surprising that α-amylase treatment had no effect on establishment of symbiosis here
and in a previous study [26]. Indeed, α-linked polysaccharides and L-rhamnose do not seem to be crucial in establishment of the cnidarian–dinoflagellate symbiosis. TRYPSIN TREATMENT Trypsin
is a protease that cleaves peptide bonds on the C-terminal side of lysine and arginine amino acid residues. It has been used widely on Symbiodiniaceae [18, 26, 30] to examine the
contribution of symbiont cell wall or surface protein moieties to host cell invasion. Previous studies have shown that homologous symbiont colonisation success was negatively affected by
trypsin treatment in _E. diaphana_ from Taiwan [18] and larvae of the coral _F. scutaria_ [26]. Conversely, an experiment on _A. tenuis_ larvae in which glycoconjugates of algal surface
glycoproteins were digested with trypsin resulted in a super-infection by _C. goreaui_, one of the compatible symbionts during the larval stage of this coral species [29]. In our study,
trypsin treatment negatively influenced colonisation by the homologous _B. minutum_ but enhanced uptake of _C. goreaui_, resulting in a super-infection of the anemones (Fig. 5 and Table 3).
A specific configuration of glycoproteins might, on one hand, ensure the recognition of _C. goreaui_ as a compatible partner; on the other hand, it might be the crucial factor that regulates
the uptake of a symbiont that, although compatible, is not the homologous type of _E. diaphana_ from the GBR. The alteration of these glycan structures, besides altering the establishment
of symbiosis with compatible symbionts, might also allow _C. goreaui_ to more easily invade the host by avoiding the host mechanism of minimizing the uptake of heterologous algae.
CONCORDANCES AND DIFFERENCES BETWEEN THE GLYCOME ANALYTICAL METHODS Our multi-technology approach to characterize the glycomes of differently compatible symbionts is the first of its kind. A
high degree of concordance between the results obtained from HPAEC-PAD, confocal and lectin array analyses emphasizes the utility of using multiple assays to explore the glycomes.
Nevertheless, some inconsistencies between the analyses were evident. The three methods rely on very different methodologies. For the HPAEC-PAD analysis, the Symbiodiniaceae cells were
lysed, AIR was extracted, hydrolysed, concentrated and analysed. For confocal microscopy, the Symbiodiniaceae cells were fixed, incubated with labelled lectins, which were then visualized by
fluorescence microscopy. For lectin array analysis, the Symbiodiniaceae cell-surface glycoproteins were extracted, concentrated, purified, biotin labelled and then exposed to the array of
immobilized lectins. Thus, three of the methods aim to extract the glycans and then modify them for identification by different means whereas the microscopy approach sends lectins into the
cell surface hoping that the glycan target is accessible. It is not surprising that some inconsistencies between sugars identified are apparent, but the overall concordance gives confidence
in these approaches. Furthermore, our lectin array—in which we used GuHCl extraction to release glycoproteins—showed marked differences among different symbiont species; differences not
reported in a previous lectin array analysis using a different extraction method [30]. Detailed inventories of the surface glycomes of compatible/incompatible symbionts will help unravel
which glycans are crucial to successful symbiosis. CONCLUSION The present study explored glycan–lectin interactions during the establishment of symbiosis between cnidarians and three species
of Symbiodiniaceae, each with a different compatibility with the model symbiotic cnidarian _E. diaphana_. We adopted a multi-step approach and profiled cell-surface molecules via nucleotide
sugar analysis, monosaccharide composition analysis, confocal microscopy and lectin array analysis. Once we had assessed the differences between the three cell-surface glycomes, we examined
the importance of selected sugar residues in the initiation of a mutualistic relationship between the three algae and three genotypes of aposymbiotic _E. diaphana_ from the GBR. D-glucose
and D-mannose were the most abundant monosaccharides of _B. minutum_, _C. goreaui_ and _F. kawagutii_. For these sugars, we envision a role as pivotal functional components of the symbiont
surface glycome, which are probably involved in the recognition between beneficial or detrimental symbionts but not necessarily associated with host discrimination among Symbiodiniaceae
species. Masking of host cell-surface lectins with L-rhamnose and the alteration of the symbiont surface with α-amylase had no influence on the establishment of symbiosis, and we therefore
assume that these sugar residues are not critical. Sialic acids and N-acetylgalactosamine showed an unclear pattern in the present analysis, which deserves further attention. Treatment of
the algal cells with trypsin changed their rate of uptake, reinforcing previous conclusions that a protein-based moiety, likely a glycoprotein, is an essential part of compatible symbiont
recognition. Intriguingly, our data also strongly support the importance of D-galactose and, in particular, β-D-galactose residues in the establishment of the cnidarian–dinoflagellate
symbiosis, and we propose a potential involvement of other sugar residues such as L-fucose, D-xylose and D-galacturonic acid in the early steps of this mutualism. REFERENCES * LaJeunesse TC,
Parkinson JE, Gabrielson PW, Jeong HJ, Reimer JD, Voolstra CR, et al. Systematic revision of Symbiodiniaceae highlights the antiquity and diversity of coral endosymbionts. Curr Biol.
2018;28:2570–80. Article CAS Google Scholar * Davy SK, Allemand D, Weis VM. Cell biology of cnidarian–dinoflagellate symbiosis. Microbiol Mol Biol Rev. 2012;76:229–61. Article CAS
Google Scholar * Nitschke MR, Craveiro SC, Brandão C, Fidalgo C, Serôdio J, Calado AJ, et al. Description of Freudenthalidium gen. nov. and Halluxium gen. nov. to formally recognize clades
Fr3 and H as genera in the family Symbiodiniaceae (Dinophyceae). J Phycol. 2020;56:923–40. Article CAS Google Scholar * Suggett DJ, Warner ME, Leggat W. Symbiotic dinoflagellate
functional diversity mediates corals survival under ecological crisis. Trends Ecol Evol. 2017;32:735–45. Article Google Scholar * Sampayo EM, Ridgway T, Bongaerts P, Hoegh-Guldberg O.
Bleaching susceptibility and mortality of corals are determined by fine-scale differences in symbiont type. Proc Natl Acad Sci USA. 2008;105:10444–9. Article CAS Google Scholar * Matthews
JL, Crowder CM, Oakley CA, Lutz A, Roessner U, Meyer E, et al. Optimal nutrient exchange and immune responses operate in partner specificity in the cnidarian–dinoflagellate symbiosis. Proc
Natl Acad Sci USA. 2017;114:13194–9. Article CAS Google Scholar * Baird AH, Guest JR, Willis BL. Systematic and biogeographical patterns in the reproductive biology of scleractinian
corals. Annu Rev Ecol Evol Syst. 2009;40:551–71. Article Google Scholar * Kirk NL, Weis VM. Animal–Symbiodinium symbioses: foundations of coral reef ecosystems. In: Hurst C (Ed). The
mechanistic benefits of microbial symbionts. Cham: Springer, Switzerland, 2016;2:269–94. * Fitt WK. The role of chemosensory behavior of Symbiodinium microadriaticum, intermediate hosts, and
host behavior in the infection of coelenterates and molluscs with zooxanthellae. Mar Biol. 1984;81:9–17. Article Google Scholar * Pasternak Z, Bachr A, Abelson A, Achituv Y. Initiation of
symbiosis between the soft coral Heteroxenia fuscescens and its zooxanthellae. Mar Ecol Prog Ser. 2004;279:113–6. Article Google Scholar * Fitt WK, Trench RK. Endocytosis of the symbiotic
dinoflagellate Symbiodinium microadriaticum Freudenthal by endodermal cells of the scyphistomae of Cassiopeia xamachana and resistance of the algae to host digestion. J Cell Sci.
1983;64:195–212. Article CAS Google Scholar * Wakefield TS, Farmer MA, Kempf SC. Revised description of the fine structure of in situ zooxanthellae genus Symbiodinium. Biol Bull.
2000;199:76–84. Article CAS Google Scholar * Wakefield TS, Kempf SC. Development of host- and symbiont-specific monoclonal antibodies and confirmation of the origin of the symbiosome
membrane in a cnidarian–dinoflagellate symbiosis. Biol Bull. 2001;200:127–43. Article Google Scholar * Rosset SL, Oakley CA, Ferrier-Pagès C, Suggett DJ, Weis VM, Davy SK. The molecular
language of the cnidarian–dinoflagellate symbiosis. Trends Microbiol. 2021;29:320–33. * Weis VM, Davy SK, Hoegh-Guldberg O, Rodriguez-Lanetty M, Pringle JR. Cell biology in model systems as
the key to understanding corals. Trends Ecol Evol. 2008;23:369–76. Article Google Scholar * Janeway CA, Medzhitov R. Innate immune recognition. Annu Rev Immunol. 2002;20:197–216. Article
CAS Google Scholar * Markell DA, Trench RK, Iglesias-Prieto R. Macromolecules associated with the cell walls of symbiotic dinoflagellates. Symbiosis 1992;12:19–31. * Lin KL, Wang JT, Fang
LS. Participation of glycoproteins on zooxanthellal cell walls in the establishment of a symbiotic relationship with the sea anemone, Aiptasia pulchella. Zool Stud. 2000;39:172–8. CAS
Google Scholar * Kvennefors EC, Leggat W, Hoegh-Guldberg O, Degnan BM, Barnes AC. An ancient and variable mannose-binding lectin from the coral Acropora millepora binds both pathogens and
symbionts. Dev Comp Immunol. 2008;32:1582–92. Article CAS Google Scholar * Kuniya N, Jimbo M, Tanimoto F, Yamashita H, Koike K, Harii S, et al. Possible involvement of Tachylectin-2-like
lectin from Acropora tenuis in the process of Symbiodinium acquisition. Fish Sci. 2015;81:473–83. Article CAS Google Scholar * Jimbo M, Yanohara T, Koike K, Koike K, Sakai R, Muramoto K,
et al. The D-galactose-binding lectin of the octocoral Sinularia lochmodes: characterization and possible relationship to the symbiotic dinoflagellates. Comp Biochem Physiol B Biochem Mol
Biol. 2000;125:227–36. Article CAS Google Scholar * Jimbo M, Yamashita H, Koike K, Sakai R, Kamiya H. Effects of lectin in the scleractinian coral Ctenactis echinata on symbiotic
zooxanthellae. Fish Sci. 2010;76:355–63. Article CAS Google Scholar * Helenius A, Aebi M. Intracellular functions of N-linked glycans. Science. 2001;291:2364–9. Article CAS Google
Scholar * Tivey TR, Parkinson JE, Mandelare PE, Adpressa DA, Peng W, Dong X, et al. N-linked surface glycan biosynthesis, composition, inhibition, and function in cnidarian–dinoflagellate
symbiosis. Micro Ecol. 2020;80:223–36. Article CAS Google Scholar * Logan DD, LaFlamme AC, Weis VM, Davy SK. Flow-cytometric characterization of the cell-surface glycans of symbiotic
dinoflagellates (Symbiodinium spp.). J Phycol. 2010;46:525–33. Article CAS Google Scholar * Wood-Charlson EM, Hollingsworth LL, Krupp DA, Weis VM. Lectin/glycan interactions play a role
in recognition in a coral/dinoflagellate symbiosis. Cell Microbiol. 2006;8:1985–93. Article CAS Google Scholar * Tortorelli G, Belderok R, Davy SK, McFadden GI, van Oppen MJH. Host
genotypic effect on algal symbiosis establishment in the coral model, the anemone Exaiptasia diaphana, from the Great Barrier Reef. Front Mar Sci. 2020;6:833. Article Google Scholar *
Dungan AM, Hartman LM, Tortorelli G, Belderok R, Lamb AM, Pisan L, et al. Exaiptasia diaphana from the Great Barrier Reef: a valuable resource for coral symbiosis research. Symbiosis.
2020;6:1–2. Google Scholar * Bay LK, Cumbo VR, Abrego D, Kool JT, Ainsworth TD, Willis BL. Infection dynamics vary between Symbiodinium types and cell surface treatments during
establishment of endosymbiosis with coral larvae. Diversity. 2011;3:356–74. Article CAS Google Scholar * Parkinson JE, Tivey TR, Mandelare PE, Adpressa DA, Loesgen S, Weis VM. Subtle
differences in symbiont cell surface glycan profiles do not explain species-specific colonization rates in a model cnidarian-algal symbiosis. Front Microbiol. 2018;9:842. Article Google
Scholar * Rautengarten C, Heazlewood JL, Ebert B. Profiling cell wall monosaccharides and nucleotide-sugars from plants. Curr Protoc Plant Biol. 2019;4:e20092. Article CAS Google Scholar
* Rautengarten C, Ebert B, Moreno I, Temple H, Herter T, Link B, et al. The Golgi localized bifunctional UDP-rhamnose/UDP-galactose transporter family of Arabidopsis. Proc Natl Acad Sci
USA. 2014;111:11563–8. Article CAS Google Scholar * Rautengarten C, Ebert B, Liu L, Stonebloom S, Smith-Moritz AM, Pauly M, et al. The Arabidopsis Golgi-localized GDP-L-fucose transporter
is required for plant development. Nat Commun. 2016;7:1–10. Article Google Scholar * Albano RM, Mourão PA. Isolation, fractionation, and preliminary characterization of a novel class of
sulfated glycans from the tunic of Styela plicata (Chordata tunicata). J Biol Chem. 1986;261:758–65. Article CAS Google Scholar * Levin RA, Suggett DJ, Nitschke MR, van Oppen MJH,
Steinberg PD. Expanding the Symbiodinium (Dinophyceae, Suessiales) toolkit through protoplast technology. J Eukaryot Microbiol. 2017;64:588–97. Article CAS Google Scholar * Matthews JL,
Sproles AE, Oakley CA, Grossman AR, Weis VM, Davy SK. Menthol-induced bleaching rapidly and effectively provides experimental aposymbiotic sea anemones (Aiptasia sp.) for symbiosis
investigations. J Exp Biol. 2016;219:306–10. PubMed Google Scholar * R Core Team. R: a language and environment for statistical computing. 2013; 201. * Wickham H, Averick M, Bryan J, Chang
W, McGowan L, François R, et al. Welcome to the Tidyverse. J Open Source Softw. 2019;4:1686. Article Google Scholar * Vidal-Dupiol J, Adjeroud M, Roger E, Foure L, Duval D, Mone Y, et al.
Coral bleaching under thermal stress: putative involvement of host/symbiont recognition mechanisms. BMC Physiol. 2009;9:1–6. Article Google Scholar * Koike K, Jimbo M, Sakai R, Kaeriyama
M, Muramoto K, Ogata T, et al. Octocoral chemical signaling selects and controls. Biol Bull. 2004;207:80–6. Article CAS Google Scholar * Jimbo M, Koike K, Sakai R, Muramoto K, Kamiya H.
Cloning and characterization of a lectin from the octocoral Sinularia lochmodes. Biochem Biophys Res Commun. 2005;330:157–62. Article CAS Google Scholar * Jimbo M, Suda Y, Koike K,
Nakamura-Tsuruta S, Kominami J, Kamei M, et al. Possible involvement of glycolipids in lectin-mediated cellular transformation of symbiotic microalgae in corals. J Exp Mar Bio Ecol.
2013;439:129–35. Article CAS Google Scholar * Takeuchi R, Jimbo M, Tanimoto F, Iijima M, Yamashita H, Suzuki G, et al. N-acetyl-D-glucosamine-binding lectin in Acropora tenuis attracts
specific Symbiodiniaceae cell culture strains. Mar Drugs. 2021;19:146. Article CAS Google Scholar Download references ACKNOWLEDGEMENTS This research was funded by the Australian
Government through the Australian Research Council Discovery Project DP160101539 and DP210100639. GT acknowledges receipt of the University of Melbourne International Research Scholarship
and Fee Remission Scholarship, and the Botany Foundation at the University of Melbourne for awarding the Ethel McLennan prize that supported Symbiodiniaceae monosaccharide and lectin array
analyses. GIMcF and MJHvO acknowledge Australian Research Council Discovery Grant DP160101539. MJHvO acknowledges Australian Research Council Laureate Fellowship FL180100036. BE acknowledges
an ARC Future Fellowship Award FT160100276, Discovery Grant DP180102630 and support from the University of Melbourne Botany Foundation. CR acknowledges the financial aid of an Albert
Shimmins COVID-19 support fund. We thank Joshua Heazlewood and Alfie Hao for the sugar nucleotide and the monosaccharide component analyses. We thank Virginia Weis, John Parkinson, Paige
Mandelare and Kruti Patel for the support in the preparation of lectin array samples. We thank Ellie Cho from the Biological Optical Microscopy Platform (BOMP) at the University of Melbourne
for assistance with confocal microscopy. We thank Kevin Bairos-Novak, Patrick Buerger and Wing Chan for the help with bioinformatics. AUTHOR INFORMATION AUTHORS AND AFFILIATIONS * School of
Biosciences, The University of Melbourne, Parkville, VIC, Australia Giada Tortorelli, Carsten Rautengarten, Berit Ebert, Madeleine J. H. van Oppen & Geoffrey I. McFadden * Department of
Animal, Plant & Soil Sciences, La Trobe Institute for Agriculture and Food, La Trobe University, Bundoora, VIC, Australia Antony Bacic * Biological Optical Microscopy Platform, The
University of Melbourne, Parkville, VIC, Australia Gabriela Segal * School of Biological Sciences, Victoria University of Wellington, Wellington, New Zealand Simon K. Davy * Australian
Institute of Marine Science, Townsville, QLD, Australia Madeleine J. H. van Oppen Authors * Giada Tortorelli View author publications You can also search for this author inPubMed Google
Scholar * Carsten Rautengarten View author publications You can also search for this author inPubMed Google Scholar * Antony Bacic View author publications You can also search for this
author inPubMed Google Scholar * Gabriela Segal View author publications You can also search for this author inPubMed Google Scholar * Berit Ebert View author publications You can also
search for this author inPubMed Google Scholar * Simon K. Davy View author publications You can also search for this author inPubMed Google Scholar * Madeleine J. H. van Oppen View author
publications You can also search for this author inPubMed Google Scholar * Geoffrey I. McFadden View author publications You can also search for this author inPubMed Google Scholar
CONTRIBUTIONS GT, BE, AB, SKD, MJHvO and GIMF contributed to the conceptual development and the final edited version of the manuscript. GT, GS, BE and CR conducted the experiments. GT
performed the analyses. GT, BE, MJHvO and GIMF wrote the manuscript. CORRESPONDING AUTHOR Correspondence to Giada Tortorelli. ETHICS DECLARATIONS COMPETING INTERESTS The authors declare no
competing interests. ADDITIONAL INFORMATION PUBLISHER’S NOTE Springer Nature remains neutral with regard to jurisdictional claims in published maps and institutional affiliations.
SUPPLEMENTARY INFORMATION SUPPLEMENTARY INFORMATION 1, 3 SUPPLEMENTARY INFORMATION 2, 4 RIGHTS AND PERMISSIONS OPEN ACCESS This article is licensed under a Creative Commons Attribution 4.0
International License, which permits use, sharing, adaptation, distribution and reproduction in any medium or format, as long as you give appropriate credit to the original author(s) and the
source, provide a link to the Creative Commons license, and indicate if changes were made. The images or other third party material in this article are included in the article’s Creative
Commons license, unless indicated otherwise in a credit line to the material. If material is not included in the article’s Creative Commons license and your intended use is not permitted by
statutory regulation or exceeds the permitted use, you will need to obtain permission directly from the copyright holder. To view a copy of this license, visit
http://creativecommons.org/licenses/by/4.0/. Reprints and permissions ABOUT THIS ARTICLE CITE THIS ARTICLE Tortorelli, G., Rautengarten, C., Bacic, A. _et al._ Cell surface carbohydrates of
symbiotic dinoflagellates and their role in the establishment of cnidarian–dinoflagellate symbiosis. _ISME J_ 16, 190–199 (2022). https://doi.org/10.1038/s41396-021-01059-w Download citation
* Received: 09 February 2021 * Revised: 30 June 2021 * Accepted: 05 July 2021 * Published: 20 July 2021 * Issue Date: January 2022 * DOI: https://doi.org/10.1038/s41396-021-01059-w SHARE
THIS ARTICLE Anyone you share the following link with will be able to read this content: Get shareable link Sorry, a shareable link is not currently available for this article. Copy to
clipboard Provided by the Springer Nature SharedIt content-sharing initiative