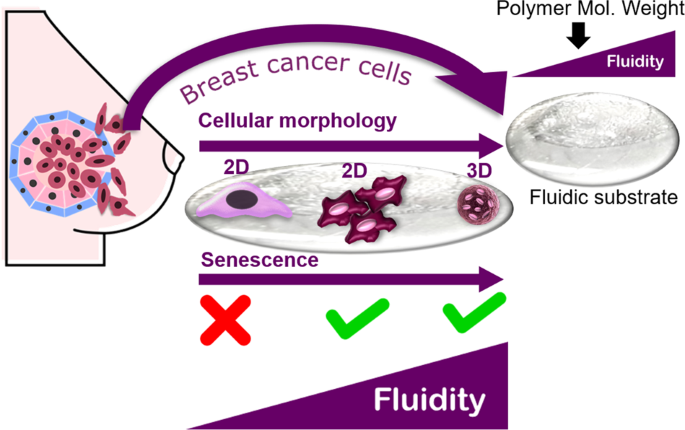
Fluidic substrate as a tool to probe breast cancer cell adaptive behavior in response to fluidity level
- Select a language for the TTS:
- UK English Female
- UK English Male
- US English Female
- US English Male
- Australian Female
- Australian Male
- Language selected: (auto detect) - EN
Play all audios:

ABSTRACT Although the roles of elastic components in breast cancer progression have been widely studied, the importance of matrix dissipative elements in regulating breast cancer behavior is
still poorly understood. In this study, we designed viscosity-tunable fluidic substrates to investigate the effects of matrix viscosity on the alteration of breast cancer cellular fate
using a hydrophobic molten polymer of poly(ε-caprolactone-_co_-D,L-lactide) [P(CL-_co_-DLLA)] with different levels of fluidity. The high- and low-fluidity substrates used in this study were
shown to behave as viscoelastic liquids at physiological temperature. A nonmetastatic breast cancer cell line (MCF-7) was cultured at the interface of the fibronectin-coated substrate, and
its behavior towards the substrate fluidity level was thoroughly characterized. Despite fibronectin-mediated cell-substrate interactions, MCF-7 cells show sensitivity to substrate fluidity
levels by forming types aggregates of different sizes and structures over time. Accordingly, MCF-7 cells were undergoing senescence on fluidic substrates, as shown by high metabolic activity
over time, suppressed proliferation ability, and positive expression of senescence markers. Moreover, senescence implies more resistance towards anticancer drug treatment. This indicates
that a fluidic substrate, as a two-dimensional synthetic matrix system, could demonstrate the importance of mechanical cues in redefining cellular function and cellular fate by changing the
viscosity of the pure substrate. You have full access to this article via your institution. Download PDF SIMILAR CONTENT BEING VIEWED BY OTHERS EFFECTS OF EXTRACELLULAR MATRIX
VISCOELASTICITY ON CELLULAR BEHAVIOUR Article 26 August 2020 ENHANCED MECHANOSENSING OF CELLS IN SYNTHETIC 3D MATRIX WITH CONTROLLED BIOPHYSICAL DYNAMICS Article Open access 10 June 2021
CELL MECHANICAL PROPERTIES OF HUMAN BREAST CARCINOMA CELLS DEPEND ON TEMPERATURE Article Open access 24 May 2021 INTRODUCTION Breast cancer is still one of the deadliest cancers in women, as
reported by the World Health Organization in 2018, with an increasing number of new cases every year. The type of treatment for breast cancer patients is commonly decided based on the
presence of certain pro-tumor proteins and hormone receptors and the proliferative ability of cancer cells. Cancer is generally formed after a series of genetic mutation events resulting in
evasion of the cell death mechanism, causing uncontrolled cell proliferation. Breast cancer progression is commonly marked by increasing extracellular matrix (ECM) stiffness due to the
deposition and cross-linking of collagen I [1]. Recently, there has been increasing evidence that the alteration of the mechanical properties of ECM in breast cancer progression is
independent of genetic mutation events [2]. Therefore, understanding the sensing ability and response of cancer cells towards mechanical cues is crucial. The ECM is the component present
within all tissues and organs that provides physical support for cellular components and initiates both biochemical and mechanical cues required for directing cellular fate, tissue
development, and maintaining homeostasis. Earlier studies have reported how mechanical cues, such as stiffness, topography, and viscoelasticity, of the ECM regulate cancer cell behavior
[3,4,5,6]. Most of the body tissues show viscoelastic behavior, including the breast [7, 8]. A viscoelastic system consists of both elastic and viscous (dissipative) elements. Recently, the
importance of dissipative elements of the viscoelastic system in regulating cell behavior has been elucidated, e.g., the spreading area and focal adhesion density of fibroblast cells changed
depending on the independently tuned-loss modulus of polyacrylamide (PAA)-based hydrogel [9]; displacement of epithelial monolayer could be driven by adjusting the loss tangent value of the
substrate [10]; and the migration behavior of liver cancer cells varied depending on the viscosity of culture medium [11]. Although the roles of elastic components, e.g., stiffness, in
breast cancer progression have been widely studied, the importance of matrix dissipative elements in regulating breast cancer behavior is still poorly understood. Patient elastography showed
that malignant breast tumors were more viscous or fluidic than benign lesions in breasts, and the rate of stress relaxation is reported to be more altered than the magnitude of the elastic
modulus in breast tumors [9]. These examples show the physiological relevance of matrix dissipative elements in breast cancer progression. Previously, many works have been conducted to mimic
the interaction between cells and the viscoelasticity of the ECM in vivo by using 2D or 3D artificial ECM models, e.g., hydrogels. Synthetic polymers, such as poly dimethyl siloxane (PDMS),
PAA, and polyethylene glycol, or natural polymers, such as alginate, have been widely used to design substrates mimicking the artificial ECM, and the viscoelasticity of substrates is
commonly tuned by physical or chemical crosslinking [12]. Regardless of the various advanced systems in modeling viscoelastic substrates, whether cell responses to dissipative systems have
the same mechanism as elasticity remains questionable. Another current issue is the challenge of designing viscous systems. Over the last few years, several strategies to control the
viscosity of viscoelastic hydrogels, such as using ionically crosslinked systems; modulating polymer design by, e.g., varying molecular weight and adding steric hindrance between chains;
using dynamically controlled crosslinking; or using physical entanglements that can easily slide off depending on stress/strain, have been used [12]. Hence, there is an emerging need to
clarify the way cells respond to dissipative systems by first designing a pure viscous system. PCL, a semicrystalline FDA-approved polymer, has been widely used in the biomedical field due
to its favorable mechanical properties [13]. However, the PCL homopolymer has a melting temperature of ~60 °C, and it is known to have a slow degradation speed (>2 years) due to its
fairly high crystallinity. Meanwhile, PDLLA, a racemic mixture of L and D lactide monomers, is an amorphous polymer. The amorphous nature of PDLLA can be utilized to manipulate the
crystallinity of PCL, resulting in a lowered melting temperature. Hence, PCL can be combined with PDLLA to create a cell culture platform with favorable polymer properties. In previous
studies, crosslinked and non-crosslinked poly(caprolactone-_co_-D,L-Lactide) [P(CL-_co_-DLLA)] were used to study how fluidity alters the cellular fate of lung cancer [14]. The viability of
cells on non-crosslinked P(CL-_co_-DLLA) was found to decrease. Interestingly, the decrease in cell viability of lung cancer cells was due to senescence instead of programmed cell death or
apoptosis [15]. Accordingly, this study was conducted to investigate the adaptive behavior of breast cancer cells in response to different levels of fluidity by using non-crosslinked
P(CL-_co_-DLLA) (Fig. 1). Different levels of substrate fluidity were obtained by modulating the molecular weight of the polymer. Moreover, the sensitivity of breast cancer cells, in terms
of cell function and fate, towards dissipative elements of the substrate was examined along with its implications in drug-based cancer treatment. EXPERIMENTAL PROCEDURE MATERIALS Toluene and
THF were purchased from Wako Pure Chemical Industries Ltd. (Osaka, Japan). Ethanol was purchased from Imazu Chemical Co., Ltd. (Tokyo, Japan). Glass coverslips (22 mm or 15 mm) were
purchased from Matsunami Glass Ind., Ltd. (Osaka, Japan). Nile red was purchased from TCI Co., Ltd. (Tokyo, Japan). MCF-7 cells were purchased from ATCC (Manassas VA, USA). DMEM,
heat-inactivated FBS, 1% L-glutamine, 1% penicillin-streptomycin, 0.25% trypsin-EDTA, fibronectin, Triton X-100, tetramethylrhodamine B isothiocyanate-conjugated phalloidin,
4′,6-diamidino-2-phenylindole (DAPI), and SA-β-Gal staining kits were purchased from Sigma-Aldrich Inc. (St. Louis, MO, USA). AlamarBlue reagent and the Quant-iT PicoGreen dsDNA Assay Kit
were purchased from Thermo Fisher Scientific (MA, USA). The cellular senescence plate assay kit- SPiDER-βGal was purchased from Dojindo Molecular Technologies, Inc. (Kumamoto, Japan).
Doxorubicin hydrochloride (DOX) was also purchased from TCI Co., Ltd. (Tokyo, Japan). SYNTHESIS AND CHARACTERIZATION OF P(CL-_CO_-DLLA) A four-branched copolymer,
poly(ε-caprolactone-_co_-D,L-lactide) [P(CL-_co_-DLLA)], was synthesized by ring-opening copolymerization of pentaerythritol using a tin octanoate catalyst as described in our earlier
reports [16, 17]. The chemical structures were confirmed by proton nuclear magnetic resonance (1H NMR) spectroscopy (JEOL, Tokyo, Japan) and the molecular weights were estimated by gel
permeation chromatography (GPC, JASCO International, Tokyo, Japan) using polystyrenes as the calibration standard and THF as the elution solvent. PREPARATION OF FLUIDIC SUBSTRATES
P(CL-_co_-DLLA) with molecular weights of 11.7 and 7.2k were dissolved in toluene at 20 and 50 wt%. The polymer solution was coated on ethanol-washed and ultraviolet (UV)-sterilized 22 mm or
15 mm glass coverslips at 3000 rpm for 2 min by spin coating (Active Spin Coater, Axel, Japan) and subjected to solvent drying at 50 °C overnight. VISUALIZATION OF FLUIDIC SUBSTRATE
FLUIDITY Fluorescently labeled P(CL-_co_-DLLA) [NR-P(CL-_co_-DLLA)] was prepared by mixing 0.5 wt% (relative to polymer weight) Nile Red (NR) with polymer. In this experiment, a PCL
homopolymer (MW: 45k) was used as a control. (NR-P(CL-_co_-DLLA) and P(CL-_co_-DLLA) were set on coverslip glass and placed inside an incubator (37 °C). The diffusion of NR dye across the
polymer boundary was observed and recorded at five different time points. Finally, recorded images were processed by using the plot profile function of ImageJ software to analyze the
diffusion of NR dye across the polymer boundary in terms of the color profile. CHARACTERIZATION OF FLUIDIC SUBSTRATES The thermal properties of the copolymers were measured by DSC (DSC6100,
Seiko Instruments, Chiba, Japan). The measurements were conducted from 0 to 120 °C at a heating rate of 5 °Cmin−1. The rheological measurement of fluidic substrates was conducted by using an
MCR 301 rheometer (Anton Paar, Germany). Fluidic substrates were placed between two parallel PP-10 plates with a gap distance of 0.2 mm. Viscoelasticity spectra (storage modulus (_G_′) and
the loss modulus (_G_″)) were obtained under frequency sweep mode with fixed strain (_γ_ = 1%) from 0.1 to 500 s−1 at a fixed temperature (37 °C). To measure the amorphous-to-crystalline
transition temperature, temperature ramp mode was performed at a fixed strain (_γ_ = 1%) and frequency (1 Hz) under a controlled temperature sweep from 25 to 37 °C at a heating rate of 0.1
°C per minute. The viscosity of different polymer solutions was measured by using microVISC™ (RheoSense, Inc., CA, USA) at a fixed temperature (37 °C). The wettability of fluidic substrates
was determined by a contact angle meter (DropMaster, Saitama, Japan). The contact angle values were taken at three different positions for each glass coverslip. CELL CULTURE MCF-7 cells were
grown in DMEM supplemented with 10% heat-inactivated FBS, 1% L-glutamine, and 1% penicillin–streptomycin. Cells were maintained at 37 °C under a humidified atmosphere of 5% CO2.
Subculturing was performed every 2–3 days with 0.25% trypsin-EDTA until use. The prepared fluidic substrates were sterilized by UV-irradiation on a UV plate for 1 h and coated with 10 μg/mL
fibronectin for 1 h at 37 °C prior to cell culture. Finally, cells were cultured at the substrate interface. ANALYSIS OF CELL FUNCTIONS ON FLUIDIC SUBSTRATES MORPHOLOGY OBSERVATION,
IMMUNOSTAINING, AND CONFOCAL MICROSCOPY For morphology observation, cells with different seeding densities (3 × 104, 5 × 104, 7 × 104, and 1 × 105 cells/cm2) were seeded on fluidic
substrates and incubated for 1–5 days. Morphology was observed by using a phase contrast microscope (ECLIPSE Ti2, Nikon, Tokyo, Japan). For confocal microscopy, 5 × 104 cells/cm2 were seeded
on fluidic substrates and incubated for 1 day. The cells were fixed with 4% paraformaldehyde for 15 min and then permeabilized using 0.1% Triton X-100 for 5 min. F-actin and nuclei of cells
were stained by tetramethylrhodamine B isothiocyanate-conjugated phalloidin and 4′,6-diamidino-2-phenylindole (DAPI), respectively. The cells were observed in scanned _z_-mode, and images
were taken by a Leica SP5 confocal laser scanning microscope (Leica, Wetzlar, Germany). CELL ACTIVITY ASSAYS Cells were seeded in triplicate at a density of 3 × 104 cells/cm2 on fluidic
substrates and glass coverslips in 24-well plate inserts for 1–5 days. The metabolic activity of cells grown on fluidic substrates and glass coverslips was assessed by using alamarBlue
reagent at different time points (days 1–5 after cell seeding). The total DNA content of cells grown on fluidic substrates and glass coverslips was quantified at different time points (days
1–5 after cell seeding) by the Quant-iT PicoGreen dsDNA Assay Kit. Both assays were performed according to the manufacturer’s instructions. Cell numbers were calculated by calculation based
on the cell DNA using a calibration curve of total cell DNA versus known numbers of cells. AlamarBlue (excitation _λ_: 570 nm/emission _λ_: 600 nm) and PicoGreen (excitation _λ_: 480
nm/emission _λ_: 520 nm) fluorescence intensities were measured using an Infinite® 200 PRO fluorescence plate reader (Tecan Trading AG, Switzerland). SENESCENCE-ASSOCIATED-Β-GALACTOSIDASE
(SA-Β-GAL) STAINING Cells were seeded at a density of 3 × 104 cells/cm2 on fluidic substrates and glass coverslips in 24-well plate inserts and cultured for different time points (1–5 days).
Then, the cells were fixed and stained for senescence-associated beta-galactosidase (SA-β-Gal) at pH 6.0 according to the manufacturer’s protocol. SA-β-Gal-stained cell images were observed
using a phase-contrast microscope (ECLIPSE Ti2, Nikon, Tokyo, Japan). Quantification of senescent cells was conducted by using the cellular senescence plate assay kit-SPiDER-βGal according
to the manufacturer’s protocol (excitation _λ_: 535 nm/emission _λ_: 580 nm). SPiDER-βGal fluorescence intensity was measured using a fluorescence plate reader (Tecan Trading AG,
Switzerland). ANTICANCER DRUG ASSAY Cells were seeded at a density of 1 × 104cells/cm2 on fluidic substrates and glass coverslips in 24-well plate inserts and incubated for 1 day. After
incubation, the cells were exposed to different concentrations (0.1–100 nM) of DOX and incubated for 3 more days. The drug response of cells was estimated by analyzing cell proliferation
using alamarBlue reagent as described before. The half maximal inhibitory concentration (IC50) of DOX for 50% cell survival was calculated by Quest Graph™ IC50 Calculator (AAT Bioquest,
Inc., CA, USA). RESULTS PHYSICAL CHARACTERIZATIONS OF FLUIDIC SUBSTRATES Polymers with two molecular weights for preparing fluidic substrates were successfully synthesized through ring
opening copolymerization with CL and DLLA by controlling the degree of polymerization. The CL/DLLA composition of 60/40 was chosen to lower the melting temperature to the physiological
temperature range (Table 1) [16, 17]. The two different levels of fluidic substrates in the form of non-crosslinked polymers are in their molten state at physiological temperature, as both
substrates have melting temperatures of ~37 °C. The melting temperature of the high-fluidity substrate is slightly higher than that of the low-fluidity substrate. The static water contact
angle of fluidic substrates prepared by spin coating was also measured as it represents the surface properties in terms of the wettability of substrates. It was observed that both fluidic
substrates in the form of thin films exhibit a hydrophobic nature (_θ_ > 90°) without any significant difference in terms of contact angle. Figure 2 illustrates the mechanical
characteristics of fluidic substrates in terms of rheology. Figure 2a, b illustrates the profile of the loss modulus/storage modulus of fluidic substrates depending on the frequency. On both
fluidic substrates, the loss modulus and storage modulus show dependency against angular frequency. For physiologically relevant frequencies (0.16 Hz), the loss modulus is higher than the
storage modulus in both low- and high-fluidity substrates (Fig. 2a, b), showing liquid-like behavior at 37 °C. One order of magnitude in modulus range difference is observed on both fluidic
substrates. The low-fluidity substrate has a higher loss modulus range than the high-fluidity substrate, owing to its higher viscosity. This difference is also reflected in the measured
viscosity of the polymer solution used in the experiment (Table 1). Moreover, both fluidic substrates show a damping loss tangent (_G_″/_G_′) larger than 1 at lower frequency, which means
that the substrates are viscosity-dominant materials. As expected, the high-fluidity substrates had a lower damping loss factor than the low-fluidity substrate, which indicated that
high-fluidity substrates have lower viscous components. The temperature dependency of the loss modulus and storage modulus was also measured at a constant frequency (1 Hz) by changing the
temperature from 22 to 37 °C. The loss modulus and storage modulus of both fluidic substrates were shown to decrease with increasing temperature, reaching the phase change from crystal to
amorphous at the intersection point (Fig. 2c). The temperature of these intersection points of both fluidic substrates, where _G_″ > _G_′, was slightly different in proportion to the
viscosity of the substrate (≈molecular weight of polymer) and was below 37 °C. This indicates that fluidic substrates behave as liquids during cell culture at 37 °C. The fluidity of
substrates was also visualized in terms of the hydrophobic dye diffusion profile (Fig. 3) across the polymer boundary. The high-fluidity substrate diffused faster than the low-fluidity
substrate, as shown by the uniform distribution of dye across the polymer boundary after 40 min at physiological temperature, while the dye was completely distributed uniformly across the
boundary in the low-fluidity substrate after 60 min. In contrast, diffusion of dye was not observed in the PCL homopolymer, representing a solid system. CELLULAR PHENOTYPES ON FLUIDIC
MATERIAL FLUIDITY LEVEL-DEPENDENT MORPHOLOGY OF CELL AGGREGATES MCF-7 cells cultured on glass coverslips coated with two types of fluidic substrates were observed with phase contrast
microscopy at 1 day and 5 days after seeding. Cells formed aggregates at day 1 after seeding on both fluidic materials; however, some cells tended to spread on low-fluidity material (Fig.
4a, b). The aggregate size in terms of area was also measured, and cells on the high-fluidity substrate clearly showed significantly smaller aggregates than the cells on the low-fluidity
substrate. Interestingly, cells on the high-fluidity material maintained their aggregated state and showed increasing size in aggregates despite continued culture until 5 days (Fig. 4c). On
the other hand, most cells on low-fluidity material showed a structured spreading morphology after 5 days of culture (Fig. 4d). Those aggregates formed on both fluidic materials also turned
out to have different structures in terms of dimensionality, as observed by confocal microscopy after 3 days of cell culture (Fig. 4e, f) and illustrated by aggregate cross section
dimensions (Fig. 4h). Cells on high-fluidity materials show more 3D aggregate structures (Fig. 4e); however, cells on low-fluidity material tend to form flattened or 2D aggregate structures
(Fig. 4f). It was clearly indicated that material fluidity governs MCF-7 cell morphology and structure. PROLIFERATION ABILITY AND METABOLIC ACTIVITY OF CELLS GROWN ON MATERIALS WITH
DIFFERENT LEVELS OF FLUIDITY MCF-7 cell proliferation and activity were observed for a period of 5 days and analyzed by using DNA content analysis and metabolic activity assessment. A
relative increase in cell number was followed by a decrease in the metabolic activity of fluidic substrates (Fig. 5a, b). On the high-fluidity substrate, cell number increased until day 3
and then did not significantly increase until day 5. However, on the low-fluidity substrate, cells show obvious cell growth during the culture period. This result was completely opposite to
that of the cells grown on glass substrate, which showed increasing cell growth over time. In terms of metabolic activity, on both fluidic substrates and glass (control) substrates,
increasing metabolic activity was observed from day 1 to day 5. However, on high-fluidity substrates, the metabolic activity of cells tends to be suppressed compared with that of cells on
low-fluidity substrates. These results suggested that material fluidity has an impact on both the proliferation ability and metabolic activity of cells. FLUIDITY OF MATERIAL PROMOTES
SENESCENCE OF BREAST CANCER CELLS The senescence induction of MCF-7 cells on fluidic materials was also examined qualitatively and quantitatively based on the expression of the commonly used
senescence expression marker senescence-associated-β-galactosidase (SA-β-Gal), which reflects the lysosomal activity of senescent cells (Fig. 6). Qualitative and quantitative expression of
SA-β-Gal in cells during the culture period was analyzed. For the colorimetric staining, senescence induction on both fluidic materials was observed over the cell culture period, as
indicated by the green–blue color. Senescence seemed to begin to occur at day 1 after cell seeding on both fluidic materials (Fig. 6b, c). The increase in senescence expression was also
observed upon a longer cell culture period, at day 5 of cell culture (Fig. 6h, i). On the glass (control) substrate, slight expression of SA-β-Gal was also detected at day 5 of cell culture.
This occurred due to the very confluent state of the cells, indicating stress-induced senescence. On fluidic substrates, senescence began from the center part of the cellular aggregates and
then gradually spread to the whole aggregate. Based on the quantitative expression of SA-β-Gal, an increase in the SA-β-Gal marker was also observed from day 1 to day 3 of cell culture (see
Fig. S4). CELL RESPONSE TO ANTICANCER DRUG TREATMENT ON FLUIDIC MATERIAL After examining the senescence effect, the drug sensitivity of MCF-7 cells to fluidic materials was also assessed
(Fig. 7). Cells on high-fluidity material tend to show higher drug resistance after anticancer drug treatment (doxorubicin, DOX) than the cells grown on low-fluidity material. The drug
sensitivity of cells is reflected by the IC50 value of DOX, which varies with different fluidic materials (Fig. 7b). Cells on high-fluidity substrates were shown to form three-dimensional
aggregates; therefore, the efficiency of DOX in killing breast cancer cells was also decreasing. Interestingly, the viability of cells grown on fluidic substrates after anticancer drug
treatment with the highest concentration (100 nM) remained 10–20%, and the level of fluidity might be strongly involved in this phenomenon. DISCUSSION The simplified model of the matrix used
in this study to demonstrate the adaptation behavior of breast cancer cells when sensing the viscosity of a material is a fluidic substrate, a hydrophobic polymer in its molten state coated
with fibronectin, a major protein component of the basal membrane that mediates cell attachment on the ECM [4, 6]. Fibronectin was used instead of the other basal membrane protein because
Charrier et al. [9] reported that fibronectin functionalization specifically mediates the viscosity sensing of fibroblast cells on linear PAA gel. Moreover, they revealed the importance of
the ligand dependence of substrate viscosity-sensing ability by showing that fibroblast cells were able to attach only on the viscous component of gel functionalized with fibronectin [9].
How breast cancer cells adapt upon sensing different levels of viscosity was also investigated by using a fluidic substrate. A low-fluidity substrate and a high-fluidity substrate were
prepared by controlling the molecular weight of the polymer. Here, ‘fluidic’ is used to generalize the dominant dissipative properties of the substrate and distinguish these substrates from
solids at 37 °C because they can diffuse like liquids (Fig. 2). The molten state of the polymer at physiological temperature could be obtained by optimizing the DLLA composition to increase
the amorphous region of the copolymer, as reported in a previous study [16, 17]. Fluidic substrates were prepared by using the spin-coating technique, which can produce thin films with
uniform thickness and flat surfaces [18]. Both high- and low-fluidity substrates are hydrophobic with a slight difference in contact angle, owing to the hydrophobic nature of P(CL-_co_-DLLA)
and the uniformly flat film prepared by spin coating; therefore, the effect of the surface chemical and structural properties of the substrate on cell behavior could be eliminated. To
eliminate the substrate thickness effect, the morphology of cell aggregates on various substrate thicknesses was first examined (see Fig. S2). Cells on 5000 rpm substrates seemed to sense
the glass substrate underneath, as few cells on high-fluidity substrates and most cells on low-fluidity substrates spread due to the too thin substrate (<1 μm). It was reported that cells
have depth-sensing ability; for example, hMSCs could sense a 3.4 μm maximum depth of thin matrix [19], and breast cancer cells (MCF-7, MDA-MB-231) proliferated normally on micropits with a
1.5 μm depth while showing reduced proliferation on 9 μm-depth micropits [20]. However, on substrates prepared at low spin speed (30–50 μm), cell observation is inhibited due to the
significantly increased opaqueness as the thick hydrophobic fluidic substrate minimizes its contact area in water. Finally, a medium thickness (15–25 μm) fluidic substrate was chosen, as the
morphology of cell aggregates can be clearly seen, but the substrate is thick enough for the cells not to probe to the glass substrate underneath. As both fluidic substrates behave as
viscoelastic liquids at physiological temperature, the implication of ±10 times the difference in damping loss tangent/tan δ at mechano-sensing frequency may need to be addressed. Murrell et
al. [10] first reported that epithelial cells could move along with the compliant PDMS substrate with tan _δ_ > 1 due to the substrate flow induced by tissue contraction. Chester et al.
[21] also observed the tan δ-driven migration of fibroblast cells along with weak attachment of ellipsoid cells at tan _δ_ > 1.4 and, in contrast, the mesenchymal transition of fibroblast
cells at tan _δ_ < 1.4. These results suggest that the loss tangent of fluidic substrates may also play a significant role in directing MCF-7 cell movement during aggregation. As the
fluidity of the substrate can be modulated by molecular weight, it was shown that the morphology of cell aggregates can also be simply altered by controlling the polymer molecular weight.
The ability of MCF-7 cells to sense the fluidity level of the substrate was confirmed by a significant difference in the cell aggregate area between the two types of substrates. This result
is consistent with the work of Bennett et al. [22], which also showed higher in cell area on more viscous substrates than on less viscous substrates. Moreover, Korouklis et al. [23] also
showed supporting evidence that the mature focal adhesion of cells was formed at lower substrate mobility, resulting in cell spreading. Moreover, different areas of cell aggregates may
reflect the displacement speed of the cell. However, to address the role of loss tangent during cell aggregation, in contrast to the previous reports [10, 21], MCF-7 cells cultured on the
substrate with a higher tan δ (high-fluidity substrate) showed a smaller aggregate area, which implies that cell–cell contact seemed to be inhibited despite the faster substrate movement in
comparison to low-fluidity substrate. Aggregation of cells on a fluidic substrate occurs because cells fail to completely exert their force on the easily deformed substrate [16]. Upon
continuing the culture period until day 5, a clear difference in the morphology of cell aggregates on both substrates could be seen, as cells seemed to coalesce into each other on the
low-fluidity substrate and formed larger spheroid-like aggregates on the high-fluidity substrate. In a previous study conducted by Zheng et al. [24], epithelial cells were found to coalesce
on viscoelastic substrates due to the motion of the substrate in regard to the viscous part of the substrate. However, the coalescence of cells observed on the low-fluidity substrate was
also found to be depend on seeding density, as cell culture performed on the low-fluidity substrate at lower seeding density resulted in the formation of cell aggregates alone (see Fig. S5).
Another interesting result regarding the morphology of cell aggregates on different levels of fluidic substrate is the formation of 3D cell aggregates on the high-fluidity substrate (Fig.
4e). In this study, cell culture was performed at the interface of the fluidic substrate, which implies that this system represents a 2D cell culture system, however, the 3D cell structure
could be obtained by simply adjusting the viscosity of the substrate. Weak cell attachment on the substrate can also be promoted by chemically modified substrates, such as ultralow adhesion
plates. However, fluidity promoted weak cell–substrate interactions generated metabolically active cell aggregates. In contrast, chemically promoted weak cell–substrate interactions by
ultralow adhesion plates generated cell aggregates with lower metabolic activity due to the necrotic zone that may be present at the center of the cell aggregate (see Fig. S3). The fluidity
level of the substrate showed a significant effect on the morphology of cell aggregates. Consequently, the cell function of MCF-7 cells was also greatly altered depending on the fluidity
level of the substrate. Despite lower metabolic activity compared with glass substrate, the increase of metabolic activity of cells on the low-fluidity substrate compared with the
high-fluidity substrate can be explained by the morphology of cell aggregates. Overall, cell metabolic activity on both fluidic substrates is less active than that on the glass substrate,
which may be explained by the weaker attachment of cells due to the fluidic nature of the substrate. This weak cell attachment on fluidic substrates may be addressed by the fact that more
focal adhesion points are formed on elastic substrates than on viscous substrates [16, 25]. On the high-fluidity substrate, cells formed 3D structured aggregates during the culture period,
which inhibited the transport of nutrition into the central part of the cell aggregate, resulting in lower metabolic activity than on the low-fluidity substrate, as cells formed 2D
structured aggregates on the low-fluidity substrate. However, despite the metabolic activity, the proliferation ability of cells on both fluidic substrates tended to be slower on the
low-fluidity substrate and almost halted on the high-fluidity substrate from day 3 of cell culture, which suggests the possibility of senescence induction. Senescent cells are basically
characterized by high metabolic activity with halted proliferation [26]. According to the expression of SA-β-Gal, the senescence of cells induced by substrate fluidity seemed to occur
earlier on the high-fluidity substrate than on the low-fluidity substrate. It is marked by the positive expression of cells on the edges of the aggregate, as cells located on the edges of
the aggregate were in direct contact with the substrate. This suggests that the induction of senescence may be controlled by the fluidity level of the substrate. The induction of senescence
may be classified as stress-induced senescence as it is caused by external factors due to cell–substrate interactions. In addition, the quantitative expression of SA-β-Gal on fluidic
substrates (see Fig. S4) implies that the fluidity level does not seem to affect the induction rate of senescence, as a similar level of SA-β-Gal expression was observed on both fluidic
substrates. The fluidity level of the substrate has a significant impact on the metabolic activity and proliferation ability of cells. However, in the case of senescence induction, only the
fluidity of the substrate matters. Finally, in terms of therapeutic implications, the high resistance of cells on the high-fluidity substrate to DOX treatment can be addressed by the
formation of 3D structured aggregates on the high-fluidity substrate, which hindered DOX transport throughout the cell aggregates. Interestingly, the viability of cells grown on fluidic
substrates after anticancer drug treatment at the highest concentration (100 nM) remained 10–20%, which may imply high survival of some populations of cells. Recently, cancer stem cell
formation in the cancer microenvironment has been widely studied, and cancer stem cells in the form of tumor spheres are commonly generated by nonadhesive (ultralow adhesion) plates. Zhang
et al. [27] reported that weak adhesion of cancer cells on PDMS substrates induces an increase in stem-cell-like subpopulations. As breast cancer cells adhere weakly to fluidic substrates
and form spheroid-like aggregates with high anticancer drug resistance, the potential of fluidic substrates as a novel platform to create a better physiological cancer model could be further
explored in the future. P(CL-_co_-DLLA) was successfully synthesized as a fluidic substrate to be used as a platform to investigate the importance of the viscosity of the matrix on
regulating breast cancer cell functions. Fluidic substrates with two different fluidity levels were prepared from P(CL-_co_-DLLA) by modulating the molecular weight, particularly the degree
of polymerization, and the melting temperature was adjusted by the addition of the D,L-lactide monomer, which decreases the crystalline region of the copolymer. Fluidic substrates have
unique properties: they are hydrophobic and behave like viscoelastic liquids. In this study, weak attachment of cells to the substrate was promoted by adjusting mechanical cues, particularly
the fluidity level of the substrate. These cell aggregates mechanically induced by the high-fluidity substrate exhibit unique characteristics compared with chemically induced cell
aggregates, as cells underwent senescence on the high-fluidity substrate instead of cell death. In this study, it can be concluded that the cellular fate of breast cancer cells could be
controlled by simply adjusting the molecular weight of the polymer. The high sensitivity of breast cancer cells to the level of fluidity was also clearly demonstrated by using a narrow range
of polymer molecular weight differences (<5000 g/mol), as significant differences in cell morphology and cell function could be observed. Based on these results, further investigation of
the threshold of substrate fluidity that induces senescence of breast cancer cells would be carried out to determine the importance of matrix mechanical properties in controlling the
dynamics of senescence. REFERENCES * Oskarsson T. Extracellular matrix components in breast cancer progression and metastasis. Breast. 2013;22:566–72.
https://doi.org/10.1016/j.breast.2013.07.012. Article Google Scholar * Lee J, Chaudhuri O. Regulation of breast cancer progression by extracellular matrix mechanics: insights from 3D
culture models. ACS Biomater Sci Eng.2018;4:302–13. https://doi.org/10.1021/acsbiomaterials.7b00071. Article CAS PubMed Google Scholar * Papalazarou V, Salmeron-Sanchez M, Machesky LM.
Tissue engineering the cancer microenvironment-challenges and opportunities. Biophys Rev. 2018;10:1695–711. https://doi.org/10.1007/s12551-018-0466-8. Article PubMed PubMed Central Google
Scholar * Walker C, Mojares E, del Río Hernández A. Role of extracellular matrix in development and cancer progression. Int J Mol Sci. 2018;19:3028 https://doi.org/10.3390/ijms19103028.
Article CAS PubMed Central Google Scholar * Muncie JM, Weaver VM. The physical and biochemical properties of the extracellular matrix regulate cell fate. Curr Top Dev Biol.
2018;130:1–37. https://doi.org/10.1016/bs.ctdb.2018.02.002. Article PubMed PubMed Central Google Scholar * Frantz C, Stewart KM, Weaver VM. The extracellular matrix at a glance. J Cell
Sci. 2010;123:4195–200. https://doi.org/10.1242/jcs.023820. Article CAS PubMed PubMed Central Google Scholar * Devi CU, Chandran RB, Vasu RM, Sood AK. Measurement of visco-elastic
properties of breast-tissue mimicking materials using diffusing wave spectroscopy. J Biomed Opt. 2007;12:034035 https://doi.org/10.1117/1.2743081. Article PubMed Google Scholar *
Chaudhuri PK, Low BC, Lim CT. Mechanobiology of tumor growth. Chem Rev. 2018;118:6499–515. https://doi.org/10.1021/acs.chemrev.8b00042. Article CAS PubMed Google Scholar * Charrier EE,
Pogoda K, Wells RG, Janmey PA. Control of cell morphology and differentiation by substrates with independently tunable elasticity and viscous dissipation. Nat Commun. 2018;9:449
https://doi.org/10.1038/s41467-018-02906-9. Article CAS PubMed PubMed Central Google Scholar * Murrell M, Kamm R, Matsudaira P. Substrate viscosity enhances correlation in epithelial
sheet movement. Biophys J. 2011;101:297–306. https://doi.org/10.1016/j.bpj.2011.05.048. Article CAS PubMed PubMed Central Google Scholar * Gonzalez-Molina J, Zhang X, Borghesan M, da
Silva JM, Awan M, Fuller B. et al. Extracellular fluid viscosity enhances liver cancer cell mechanosensing and migration. Biomaterials . 2018;177:113–24.
https://doi.org/10.1016/j.biomaterials.2018.05.058. Article CAS PubMed Google Scholar * Cantini M, Donnelly H, Dalby MJ, Salmeron‐Sanchez M. The plot thickens: the emerging role of
matrix viscosity in cell mechanotransduction. Adv Healthcare Mater. 2019. https://doi.org/10.1002/adhm.201901259. * Poh PSP, Hege C, Chhaya MP, Balmayor ER, Foehr P, Burgkart RH, et al.
Evaluation of polycaprolactone−poly-D,L-lactide copolymer as biomaterial for breast tissue engineering. Polym Int. 2017;66:77–84. https://doi.org/10.1002/pi.5181. Article CAS Google
Scholar * Mano SS, Uto K, Aoyagi T, Ebara M. Fluidity of biodegradable substrate regulates carcinoma cell behavior: a novel approach to cancer therapy. AIMS Mater Sci. 2016;3:66–82.
https://doi.org/10.3934/matersci.2016.1.66. Article CAS Google Scholar * Mano SS, Uto K, Ebara M. Material-induced senescence (MIS): fluidity induces senescent type cell death of lung
cancer cells via insulin-like growth factor binding protein 5. Theranostics. 2017;7:4658–70. https://doi.org/10.7150/thno.20582. Article CAS PubMed PubMed Central Google Scholar * Uto
K, Mano SS, Aoyagi T, Ebara M. Substrate fluidity regulates cell adhesion and morphology on poly (ε-caprolactone)-based materials. ACS Biomater Sci Eng. 2016;2:446–53.
https://doi.org/10.1021/acsbiomaterials.6b00058. Article CAS PubMed Google Scholar * Uto K, Muroya T, Okamoto M, Tanaka H, Murase T, Ebara M, et al. Design of super-elastic biodegradable
scaffolds with longitudinally oriented microchannels and optimization of the channel size for Schwann cell migration. Sci Technol Adv Mater. 2012;13:064207.
https://doi.org/10.1088/1468-6996/13/6/064207. Article CAS PubMed PubMed Central Google Scholar * Dzhoyashvili NA, Thompson K, Gorelov AV, Rochev YA. Film thickness determines cell
growth and cell sheet detachment from spin-coated poly(N‑Isopropylacrylamide) substrates. ACS Appl Mater Interfaces. 2016;8:27564–572. https://doi.org/10.1021/acsami.6b09711. Article CAS
PubMed Google Scholar * Buxboim A, Rajagopal K, Brown AE, Discher DE. How deeply cells feel: methods for thin gels. J Condens. 2010;22:194116.
https://doi.org/10.1088/0953-8984/22/19/194116. Article CAS Google Scholar * Chaudhuri PK, Pan CQ, Low BC, Lim CT. Differential depth sensing reduces cancer cell proliferation via
rho-rac-regulated invadopodia. ACS Nano. 2017;11:7336–48. https://doi.org/10.1021/acsnano.7b03452. Article CAS PubMed Google Scholar * Chester D, Kathard R, Nortey J, Nellenbach K, Brown
AC. Viscoelastic properties of microgel thin films control fibroblast modes of migration and pro-fibrotic responses. Biomaterials. 2018;185:371–82.
https://doi.org/10.1016/j.biomaterials.2018.09.012. Article CAS PubMed Google Scholar * Bennett M, Cantini M, Reboud J, Cooper JM, Roca-Cusachs P, Salmeron-Sanchez M. Molecular clutch
drives cell response to surface viscosity. PNAS. 2018;115:1192–7. https://doi.org/10.1073/pnas.1710653115. Article CAS PubMed PubMed Central Google Scholar * Kourouklis AP, Lerum RV,
Bermudez H. Cell adhesion mechanisms on laterally mobile polymer films. Biomaterials. 2014;35:4827–34. https://doi.org/10.1016/j.biomaterials.2014.02.052. Article CAS PubMed Google
Scholar * Zheng JY, Han SP, Chiu YJ, Yip AK, Boichat N, Zhu SW, et al. Epithelial monolayers coalesce on a viscoelastic substrate through redistribution of vinculin. Biophys J.
2017;113:1585–98. https://doi.org/10.1016/j.bpj.2017.07.027. Article CAS PubMed PubMed Central Google Scholar * Bell S, Terentjev EM. Focal adhesion kinase: the reversible molecular
mechanosensor. Biophys J. 2017;112:2439–50. https://doi.org/10.1016/j.bpj.2017.04.048. Article CAS PubMed PubMed Central Google Scholar * Gorgoulis V, Adams PD, Alimonti A, Bennett DC,
Bischof O, Bishop C, et al. Cellular senescence: defining a path forward. Cell. 2019;179:813–27. https://doi.org/10.1016/j.cell.2019.10.005. Article CAS PubMed Google Scholar * Zhang W,
Choi DS, Nguyen YH, Chang J, Qin L. Studying cancer stem cell dynamics on PDMS surfaces for microfluidics device design. Sci Rep. 2013;3:2332. https://doi.org/10.1038/srep02332. Article
PubMed PubMed Central Google Scholar Download references ACKNOWLEDGEMENTS The authors would like to express their gratitude to the Grants-in-Aid for Scientific Research (B) (19H04476)
from the Ministry of Education, Culture, Sports, Science and Technology (MEXT) Japan. The authors are grateful to Allan S. Hoffman (University of Washington) for continued and valuable
discussion. AUTHOR INFORMATION AUTHORS AND AFFILIATIONS * Graduate School of Pure and Applied Sciences, University of Tsukuba, 1-1-1 Tennodai, Tsukuba, Ibaraki, 305-8577, Japan Mazaya
Najmina & Mitsuhiro Ebara * International Center for Materials Nanoarchitectonics (WPI-MANA), National Institute for Materials Science (NIMS), 1-1 Namiki, Tsukuba, Ibaraki, 305-0044,
Japan Mazaya Najmina, Koichiro Uto & Mitsuhiro Ebara * Graduate School of Industrial Science and Technology, Tokyo University of Science, 6-3-1 Niijuku, Katsushika-ku, Tokyo, 125-8585,
Japan Mitsuhiro Ebara Authors * Mazaya Najmina View author publications You can also search for this author inPubMed Google Scholar * Koichiro Uto View author publications You can also
search for this author inPubMed Google Scholar * Mitsuhiro Ebara View author publications You can also search for this author inPubMed Google Scholar CORRESPONDING AUTHOR Correspondence to
Mitsuhiro Ebara. ETHICS DECLARATIONS CONFLICT OF INTEREST The authors declare that they have no conflict of interest. ADDITIONAL INFORMATION PUBLISHER’S NOTE Springer Nature remains neutral
with regard to jurisdictional claims in published maps and institutional affiliations. SUPPLEMENTARY INFORMATION SUPPLEMENTAL INFORMATION RIGHTS AND PERMISSIONS Reprints and permissions
ABOUT THIS ARTICLE CITE THIS ARTICLE Najmina, M., Uto, K. & Ebara, M. Fluidic substrate as a tool to probe breast cancer cell adaptive behavior in response to fluidity level. _Polym J_
52, 985–995 (2020). https://doi.org/10.1038/s41428-020-0345-6 Download citation * Received: 20 January 2020 * Revised: 18 March 2020 * Accepted: 23 March 2020 * Published: 22 April 2020 *
Issue Date: August 2020 * DOI: https://doi.org/10.1038/s41428-020-0345-6 SHARE THIS ARTICLE Anyone you share the following link with will be able to read this content: Get shareable link
Sorry, a shareable link is not currently available for this article. Copy to clipboard Provided by the Springer Nature SharedIt content-sharing initiative