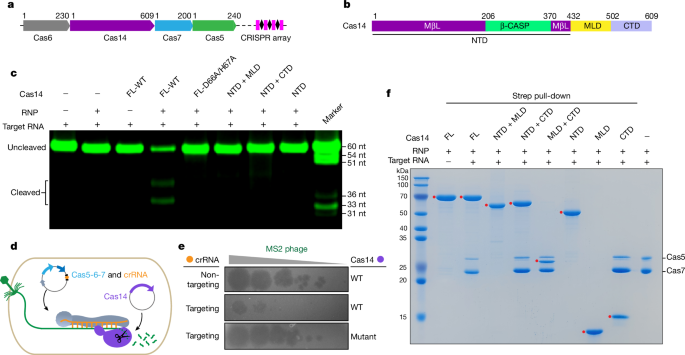
Structural basis for the activity of the type vii crispr–cas system
- Select a language for the TTS:
- UK English Female
- UK English Male
- US English Female
- US English Male
- Australian Female
- Australian Male
- Language selected: (auto detect) - EN
Play all audios:

ABSTRACT The newly identified type VII CRISPR–Cas candidate system uses a CRISPR RNA-guided ribonucleoprotein complex formed by Cas5 and Cas7 proteins to target RNA1. However, the RNA
cleavage is executed by a dedicated Cas14 nuclease, which is distinct from the effector nucleases of the other CRISPR–Cas systems. Here we report seven cryo-electron microscopy structures of
the Cas14-bound interference complex at different functional states. Cas14, a tetrameric protein in solution, is recruited to the Cas5–Cas7 complex in a target RNA-dependent manner. The
N-terminal catalytic domain of Cas14 binds a stretch of the substrate RNA for cleavage, whereas the C-terminal domain is primarily responsible for tethering Cas14 to the Cas5–Cas7 complex.
The biochemical cleavage assays corroborate the captured functional conformations, revealing that Cas14 binds to different sites on the Cas5–Cas7 complex to execute individual cleavage
events. Notably, a plugged-in arginine of Cas7 sandwiched by a C-shaped clamp of C-terminal domain precisely modulates Cas14 binding. More interestingly, target RNA cleavage is altered by a
complementary protospacer flanking sequence at the 5′ end, but not at the 3′ end. Altogether, our study elucidates critical molecular details underlying the assembly of the interference
complex and substrate cleavage in the type VII CRISPR–Cas system, which may help rational engineering of the type VII CRISPR–Cas system for biotechnological applications. Access through your
institution Buy or subscribe This is a preview of subscription content, access via your institution ACCESS OPTIONS Access through your institution Access Nature and 54 other Nature
Portfolio journals Get Nature+, our best-value online-access subscription $29.99 / 30 days cancel any time Learn more Subscribe to this journal Receive 51 print issues and online access
$199.00 per year only $3.90 per issue Learn more Buy this article * Purchase on SpringerLink * Instant access to full article PDF Buy now Prices may be subject to local taxes which are
calculated during checkout ADDITIONAL ACCESS OPTIONS: * Log in * Learn about institutional subscriptions * Read our FAQs * Contact customer support SIMILAR CONTENT BEING VIEWED BY OTHERS A
TYPE III-ASSOCIATED CAS6 FUNCTIONS AS A NEGATIVE REGULATOR OF TYPE I-B CRISPR-CAS SYSTEM IN _THERMUS THERMOPHILUS_ Article Open access 23 May 2025 INSIGHTS INTO THE COMPACT CRISPR–CAS9D
SYSTEM Article Open access 12 March 2025 PROGRAMMABLE RNA TARGETING WITH THE SINGLE-PROTEIN CRISPR EFFECTOR CAS7-11 Article 06 September 2021 DATA AVAILABILITY The atomic coordinates and EM
maps have been deposited in the Protein Data Bank under accession codes 8Z4L (substrate-engaged state I), 8Z4J (substrate-engaged state II), 8YHD (post-state I), 8YHE (post-state II), 8Z99
(substrate-engaged state +I), 8Z9C (substrate-engaged state II) and 8Z9E (substrate-engaged state II), and in the Electron Microscopy Data Bank under the corresponding accession codes
EMD-39767, EMD-39766, EMD-39287, EMD-39288, EMD-39857, EMD-39859 and EMD-39861. PDB accessions 2I7V, 3X1L, 4XWT, 6IFU and 6V4X were used for structure analysis. Uncropped gels and images are
provided in Supplementary Figs. 1–3. Source data are provided with this paper. REFERENCES * Altae-Tran, H. et al. Uncovering the functional diversity of rare CRISPR-Cas systems with deep
terascale clustering. _Science_ 382, eadi1910 (2023). Article CAS PubMed PubMed Central Google Scholar * Hille, F. et al. The biology of CRISPR-Cas: backward and forward. _Cell_ 172,
1239–1259 (2018). Article CAS PubMed Google Scholar * Makarova, K. S. et al. Evolutionary classification of CRISPR–Cas systems: a burst of class 2 and derived variants. _Nat. Rev.
Microbiol._ 18, 67–83 (2020). Article CAS PubMed Google Scholar * Liu, T. Y. & Doudna, J. A. Chemistry of class 1 CRISPR-Cas effectors: binding, editing, and regulation. _J. Biol.
Chem._ 295, 14473–14487 (2020). Article CAS PubMed PubMed Central Google Scholar * You, L. et al. Structure studies of the CRISPR-Csm complex reveal mechanism of co-transcriptional
interference. _Cell_ 176, 239–253.e16 (2019). Article CAS PubMed Google Scholar * Jia, N. et al. Type III-A CRISPR-Cas Csm complexes: assembly, periodic RNA cleavage, DNase activity
regulation, and autoimmunity. _Mol. Cell_ 73, 264–277.e65 (2019). Article CAS PubMed Google Scholar * Yu, G. et al. Structure and function of a bacterial type III–E CRISPR-Cas7–11
complex. _Nat. Microbiol._ 7, 2078–2088 (2022). Article CAS PubMed Google Scholar * Wang, S., Guo, M., Zhu, Y., Lin, Z. & Huang, Z. Cryo-EM structure of the type III-E CRISPR-Cas
effector gRAMP in complex with TPR-CHAT. _Cell Res._ 32, 1128–1131 (2022). Article CAS PubMed PubMed Central Google Scholar * Strecker, J. et al. RNA-activated protein cleavage with a
CRISPR-associated endopeptidase. _Science_ 378, 874–881 (2022). Article CAS PubMed PubMed Central ADS Google Scholar * Liu, X. et al. Target RNA activates the protease activity of
Craspase to confer antiviral defense. _Mol. Cell_ 82, 4503–4518.e8 (2022). Article CAS PubMed Google Scholar * Kato, K. et al. RNA-triggered protein cleavage and cell growth arrest by
the type III-E CRISPR nuclease-protease. _Science_ 378, 882–889 (2022). Article CAS PubMed PubMed Central ADS Google Scholar * Hu, C. et al. Craspase is a CRISPR RNA-guided,
RNA-activated protease. _Science_ 377, 1278–1285 (2022). Article CAS PubMed PubMed Central ADS Google Scholar * Wang, X. et al. Target RNA-guided protease activity in type III-E
CRISPR-Cas system. _Nucleic Acids Res._ 50, 12913–12923 (2022). Article CAS PubMed PubMed Central ADS Google Scholar * Sofos, N. et al. Structures of the Cmr-β complex reveal the
regulation of the immunity mechanism of type III-B CRISPR-Cas. _Mol Cell._ 79, 741–757.e7 (2020). Article CAS PubMed Google Scholar * Callebaut, I., Moshous, D., Mornon, J. P. & de
Villartay, J. P. Metallo-β-lactamase fold within nucleic acids processing enzymes: the β-CASP family. _Nucleic Acids Res._ 30, 3592–3601 (2002). Article CAS PubMed PubMed Central Google
Scholar * Moshous, D. et al. Artemis, a novel DNA double-strand break repair/V(D)J recombination protein, is mutated in human severe combined immune deficiency. _Cell_ 105, 177–186 (2001).
Article CAS PubMed Google Scholar * Phung, D. K. et al. RNA processing machineries in Archaea: the 5′–3′ exoribonuclease aRNase J of the β-CASP family is engaged specifically with the
helicase ASH-Ski2 and the 3′–5′ exoribonucleolytic RNA exosome machinery. _Nucleic Acids Res._ 48, 3832–3847 (2020). Article CAS PubMed PubMed Central Google Scholar * Zhao, Y. et al.
Structural insights into catalysis and dimerization enhanced exonuclease activity of RNase J. _Nucleic Acids Res._ 43, 5550–5559 (2015). Article CAS PubMed PubMed Central Google Scholar
* Sun, Y. et al. Structure of an active human histone pre-mRNA 3′-end processing machinery. _Science_ 367, 700–703 (2020). Article CAS PubMed PubMed Central ADS Google Scholar *
Jumper, J. et al. Highly accurate protein structure prediction with AlphaFold. _Nature_ 596, 583–589 (2021). Article CAS PubMed PubMed Central ADS Google Scholar * Wang, Z., Fast, W.,
Valentine, A. M. & Benkovic, S. J. Metallo-β-lactamase: structure and mechanism. _Curr. Opin. Chem. Biol._ 3, 614–622 (1999). Article CAS PubMed Google Scholar * Yang, X. C.,
Sullivan, K. D., Marzluff, W. F. & Dominski, Z. Studies of the 5′ exonuclease and endonuclease activities of CPSF-73 in histone pre-mRNA processing. _Mol. Cell. Biol._ 29, 31–42 (2009).
Article PubMed Google Scholar * Xiao, Y. et al. Structure basis for directional R-loop formation and substrate handover mechanisms in type I CRISPR-Cas system. _Cell_ 170, 48–60.e11
(2017). Article CAS PubMed PubMed Central Google Scholar * O’Brien, R. E. et al. Structural basis for assembly of non-canonical small subunits into type I-C Cascade. _Nat. Commun._ 11,
5931 (2020). Article PubMed PubMed Central ADS Google Scholar * Hu, C. et al. Allosteric control of type I–A CRISPR–Cas3 complexes and establishment as effective nucleic acid detection
and human genome editing tools. _Mol. Cell_ 82, 2754–2768.e5 (2022). Article CAS PubMed PubMed Central Google Scholar * Schwartz, E. A. et al. Structural rearrangements allow nucleic
acid discrimination by type I-D Cascade. _Nat. Commun._ 13, 2829 (2022). Article CAS PubMed PubMed Central ADS Google Scholar * O’Brien, R. E. et al. Structural snapshots of R-loop
formation by a type I-C CRISPR Cascade. _Mol. Cell_ 83, 746–758.e5 (2023). Article PubMed PubMed Central Google Scholar * Goswami, H. N., Rai, J., Das, A. & Li, H. Molecular
mechanism of active Cas7-11 in processing CRISPR RNA and interfering target RNA. _eLife_ 11, e81678 (2022). Article CAS PubMed PubMed Central Google Scholar * Mathy, N. et al. _Bacillus
subtilis_ ribonucleases J1 and J2 form a complex with altered enzyme behaviour. _Mol. Microbiol._ 75, 489–498 (2010). Article CAS PubMed Google Scholar * Punjani, A., Rubinstein, J. L.,
Fleet, D. J. & Brubaker, M. A. cryoSPARC: algorithms for rapid unsupervised cryo-EM structure determination. _Nat. Methods_ 14, 290–296 (2017). Article CAS PubMed Google Scholar *
Mirdita, M. et al. ColabFold: making protein folding accessible to all. _Nat. Methods_ 19, 679–682 (2022). Article CAS PubMed PubMed Central Google Scholar * Meng, E. C. et al. UCSF
ChimeraX: tools for structure building and analysis. _Protein Sci._ 32, e4792 (2023). Article CAS PubMed PubMed Central Google Scholar * Emsley, P., Lohkamp, B., Scott, W. G. &
Cowtan, K. Features and development of Coot. _Acta Crystallogr. D_ 66, 486–501 (2010). Article CAS PubMed PubMed Central ADS Google Scholar * Afonine, P. V. et al. Real-space
refinement in PHENIX for cryo-EM and crystallography. _Acta Crystallogr. D_ 74, 531–544 (2018). Article CAS ADS Google Scholar * Williams, C. J. et al. MolProbity: more and better
reference data for improved all-atom structure validation. _Protein Sci._ 27, 293–315 (2018). Article CAS PubMed Google Scholar * Liebschner, D. et al. Macromolecular structure
determination using X-rays, neutrons and electrons: recent developments in Phenix. _Acta Crystallogr. D_ 75, 861–877 (2019). Article CAS ADS Google Scholar Download references
ACKNOWLEDGEMENTS We thank the Center for Instrumental Analysis and Metrology of Wuhan Institute of Virology for supporting cryo-EM data acquisition and the Core Facility of Research Center
of Basic Medical Sciences in Tianjin Medical University for providing technical assistance. This work was supported by the National Natural Science Foundation of China (32322040 to H. Zhang,
22377091 to J.Y. and 32300036 to H.Y.), the Natural Science Foundation of Tianjin Municipal Science and Technology Commission (23JCZDJC00410) and the CAS Pioneer Hundred Talents Program (to
Z.D.). AUTHOR INFORMATION Author notes * These authors contributed equally: Jie Yang, Xuzichao Li, Qiuqiu He, Xiaoshen Wang, Jingjing Tang, Tongyao Wang AUTHORS AND AFFILIATIONS * State Key
Laboratory of Experimental Hematology, Key Laboratory of Immune Microenvironment and Disease (Ministry of Education), International Joint Laboratory of Ocular Diseases (Ministry of
Education), The Province and Ministry Co-sponsored Collaborative Innovation Center for Medical Epigenetics, Tianjin Institute of Immunology, School of Basic Medical Sciences, Tianjin Medical
University, Tianjin, China Jie Yang, Xuzichao Li, Qiuqiu He, Xiaoshen Wang, Tongyao Wang, Yi Zhang, Shuqin Zhang, Zhikun Liu, Lingling Zhang, Fumeng Liao, Hang Yin & Heng Zhang * Key
Laboratory of Virology and Biosafety, Wuhan Institute of Virology, Chinese Academy of Sciences, Wuhan, China Jingjing Tang & Zengqin Deng * University of Chinese Academy of Sciences,
Beijing, China Jingjing Tang * State Key Laboratory of Virology, College of Life Sciences, Wuhan University, Wuhan, China Feiyang Yu & Haiyan Zhao * Hubei Jiangxia Laboratory, Wuhan,
China Zengqin Deng Authors * Jie Yang View author publications You can also search for this author inPubMed Google Scholar * Xuzichao Li View author publications You can also search for this
author inPubMed Google Scholar * Qiuqiu He View author publications You can also search for this author inPubMed Google Scholar * Xiaoshen Wang View author publications You can also search
for this author inPubMed Google Scholar * Jingjing Tang View author publications You can also search for this author inPubMed Google Scholar * Tongyao Wang View author publications You can
also search for this author inPubMed Google Scholar * Yi Zhang View author publications You can also search for this author inPubMed Google Scholar * Feiyang Yu View author publications You
can also search for this author inPubMed Google Scholar * Shuqin Zhang View author publications You can also search for this author inPubMed Google Scholar * Zhikun Liu View author
publications You can also search for this author inPubMed Google Scholar * Lingling Zhang View author publications You can also search for this author inPubMed Google Scholar * Fumeng Liao
View author publications You can also search for this author inPubMed Google Scholar * Hang Yin View author publications You can also search for this author inPubMed Google Scholar * Haiyan
Zhao View author publications You can also search for this author inPubMed Google Scholar * Zengqin Deng View author publications You can also search for this author inPubMed Google Scholar
* Heng Zhang View author publications You can also search for this author inPubMed Google Scholar CONTRIBUTIONS J.Y., Z.D. and H. Zhang conceived the project. J.Y., X.L., Q.H., X.W., J.T.,
T.W., Y.Z., F.Y., L.Z. and F.L. carried out the experimental work. Data analysis was performed by J.Y., X.L., X.W., J.T., S.Z., Z.L., H.Y., H. Zhao, Z.D. and H. Zhang. H. Zhang supervised
the work. J.Y. and H. Zhang wrote the manuscript with contributions from all authors. CORRESPONDING AUTHORS Correspondence to Zengqin Deng or Heng Zhang. ETHICS DECLARATIONS COMPETING
INTERESTS The authors declare no competing interests. PEER REVIEW PEER REVIEW INFORMATION _Nature_ thanks Chunyi Hu, David Taylor and John van der Oost for their contribution to the peer
review of this work. ADDITIONAL INFORMATION PUBLISHER’S NOTE Springer Nature remains neutral with regard to jurisdictional claims in published maps and institutional affiliations. EXTENDED
DATA FIGURES AND TABLES EXTENDED DATA FIG. 1 FUNCTIONAL EXPLORATION OF THE CANDIDATE TYPE VII CRISPR–CAS SYSTEM. (A) The EMSA assay with RNP complex, catalytic dead Cas14 (D66A/H67A), or a
combination of both. The triangle indicates the increasing concentrations of proteins. Representative gels from three independent EMSA experiments are shown. (B) In vitro cleavage assay
using ssRNA, ssDNA and dsDNA as the substrate. Only the ssRNA substrate was cleaved by the combination of the RNP complex and WT Cas14. Three biological replicates were performed, and
representative gel is shown. (C) In vivo transcriptional repression assays of the type VII candidate system. BL21(DE3) cells were co-transformed with three plasmids: one encoding the
Cas5-Cas6-Cas7 with an mRFP or KanR targeting crRNA array (SpecR), one encoding WT Cas14 or D66A/H67A mutant Cas14 (AmpR), and a third encoding mRFP or KanR (KanR). The transformed cells
were induced with 0.5 mM IPTG and plated on solid LB agar containing three antibiotics and IPTG. Representative images from four independent assays are shown. (D) The percentage of
mRFP-positive colonies (left panel) and the number of colonies formed on plates containing three antibiotics (right panel) were determined by manual colony counting. Bar plots show mean ±
standard deviation (s.d.) with individual data points. P-values were calculated from four biological replicates (N = 4) using an unpaired two-tailed t-test with Welch’s corrections. (E)
Scheme of Cas14 domain truncations, related to Fig. 1f. (F) Input for the strep pull-down assay, related to Fig. 1f. The gel is representative of three biological replicates. For gel source
data, see Supplementary Figs. 1–3. Source Data EXTENDED DATA FIG. 2 STRUCTURAL FEATURES OF THE CAS5-CAS7 FILAMENT. (A) Overall structure of Cas5-Cas7 filaments in the types VII (upper panel,
this study), III-A (middle panel, PDB ID: 6IFU) and III-B (lower panel, PDB ID: 3X1L) systems. (B) Structural alignment of Cas5 in the type VII system (green) and Cmr3 in the type III-B
system (wheat, PDB ID: 3X1L). The Cas5 protein adopts a ferredoxin-like fold, similar to Csm4/Cmr3 proteins from the type III-A/B systems. Despite the structural similarities between Cas5
and its counterparts Csm4/Cmr3 of type III systems, the crucial regions of Csm4/Cmr3 responsible for the interaction with Cas10 protein are partially absent in Cas5, which is in line with
the lack of Cas10 in the type VII system. (C) Structural alignment of Cas7 in the type VII system (cyan) and Csm3 in the type III-A system (wheat, PDB ID: 6IFU). The single Cas7 unit,
structurally analogous to Csm3/Cmr4 proteins, possesses a conserved thumb-palm-finger architecture, with the thumb region flanked by the palm and finger segments from the adjacent Cas7. In
the type III systems, Csm3/Cmr4 proteins feature a catalytic loop containing an aspartic acid residue responsible for target RNA cleavage. However, the equivalent position in Cas7 of the
type VII system is occupied by an asparagine residue (Asn36), rendering Cas7 catalytically inactive. (D) Electrostatic surface representation of Cas5-Cas7 filament in the type VII system.
The crRNA-target RNA duplex binds to the positively charged channel of this filament. The EM density corresponding to the crRNA-target RNA duplex traverses through the positively charged
channel of the Cas5-Cas7 helical filament. (E) Interaction interface between the 5′-end repeat segment of crRNA and Cas5-Cas7 filament. The 5′-end repeat segment displays an S-shaped
conformation, with the -1U and (-3A)–(-6A) of the repeat sequence fully exposed, whereas -7U and -8U are buried within Cas5. EXTENDED DATA FIG. 3 STRUCTURAL FEATURES OF CAS14 DOMAINS. (A)
Size-exclusion chromatography coupled with multi-angle light scattering (SEC–MALS) analysis of Cas14 variants. The theoretical molecular weights of the tetrameric full-length Cas14 protein,
tetrameric NTD + MLD protein and the dimeric NTD protein (284 kDa, 231 kDa and 99 kDa, respectively) are consistent with their experimental results. (B) Structural alignment of the NTDs in
Cas14.1 and Cas14.3 (left). Ribbon representation of the MβL and β-CASP subdomains of NTD-Cas14.1 (right). (C) Structural alignment of the MLDs from the four Cas14 subunits (Cas14.1-14.4)
(top). Ribbon representation of the MLD (bottom). (D) Structural alignment of the CTDs from the four Cas14 subunits (Cas14.1–14.4) (left). Ribbon representation of the CTD (right). (E)
Structural comparison of CTD-Cas14 and CTD-Cas10 or Cas11 subunits in the type III CRISPR–Cas systems. Left panel: Superimposition of CTD-Cas14 (light blue) and CTD-Cas10 (white, PDB ID:
6IFU) in the type III-A system with a root mean squared deviation (r.m.s.d.) of 3.33 Å. Middle panel: Superimposition of CTD-Cas14 (light blue) and Csm2 subunit (orange, PDB ID: 6IFU) in the
type III-A system with a root mean squared deviation (r.m.s.d.) of 5.79 Å. Right panel: Superimposition of CTD-Cas14 (light blue) and Cmr5 subunit (yellow, PDB ID: 3X1L) in the type III-B
system with a root mean squared deviation (r.m.s.d.) of 5.67 Å. EXTENDED DATA FIG. 4 CAS14 SELF-ASSEMBLY IS ESSENTIAL FOR NUCLEASE ACTIVITY. (A) Structure of the Cas14 tetramer. The NTD
dimer interface and MLD tetramer interface are indicated by dashed boxes. The color scheme is the same as in Fig. 2. (B) Detailed view of the interactions between the NTDs from Cas14.1 and
Cas14.3. Key residues in the NTD dimer interface are shown in stick representation. (C) Close-up view of the tetramer interface formed by MLDs of Cas14.1–Cas14.4. The MLD, which is
dispensable for Cas14 binding to the Cas5-Cas7 complex, may serve a role in delivering the NTD and CTD to facilitate their correct orientations. (D) SEC–MALS analysis of full length-Cas14
(F447A/P454A/F458A) and NTD-Cas14 (Y388A/Y391A/R416A). The theoretical monomeric molecular masses of these two variants are 71 and 51 kDa, respectively. The experimental molecular weight of
Y388A/Y391A/R416A mutant is ~ 52 kDa, suggesting a monomer in solution. However, the F447A/P454A/F458A mutant protein is not homogeneous and exhibits a broad range of calculated molar
masses, highlighting that tetramerization is crucial for Cas14 assembly and function. (E) In vitro target RNA cleavage assay with Cas14 variants harboring mutations in the dimer and tetramer
interfaces. The gel is representative of three biological replicates. For gel source data, see Supplementary Fig. 1. EXTENDED DATA FIG. 5 INTERDOMAIN AND INTERSUBUNIT INTERACTIONS IN
COMPLEX ASSEMBLY. (A) The interdomain contacts for Cas14. The α15 and α16 helices of the CTD are tethered to the NTD through a combination of hydrophobic and polar contacts. In particular,
Lys543 of the CTD wedges between the MβL and β-CASP subdomains, while two other lysine residues, Lys540 and Lys547 of the CTD, stack against the MβL and β-CASP subdomains, respectively. The
MβL and β-CASP subdomains from Cas14.1 are highlighted in purpleblue and green, respectively. The CTD from Cas14.1 is colored in light blue. The zoomed-in view illustrates the detailed
interdomain contacts between the NTD and CTD (right panel). Key residues involved in the interdomain interaction are shown as sticks. (B) In vitro target RNA cleavage assay of WT and mutant
Cas14 proteins. The K540A/K543A/K547A mutation showed compromised nuclease activity. Representative gel image is from three biological replicates. (C) The NTD and MLD-involved contacts with
Cas7 or target RNA are indicated by black (NTD-target RNA), red (NTD-Cas7) and blue (MLD-Cas7) rectangles. (D–F) The zoomed-in view of NTD-target RNA (D), NTD-Cas7 (E) and MLD-Cas7 (F)
interaction interfaces. Key interaction residues are represented as sticks. (G) In vitro target RNA cleavage using Cas14 mutants. The gel is representative of three biological replicates.
For gel source data, see Supplementary Fig. 1. EXTENDED DATA FIG. 6 BIOCHEMICAL FEATURES AND PROPOSED CATALYTIC MECHANISMS OF CAS14. (A) Schematic representation of the crRNA and target
RNAs. The 5′-FAM labeled target RNA was used for cleavage. (B) Fluorescence mapping of the initial cleavage site. In vitro 5′-labeled RNA cleavage assay results showed that a 4-nt product
was produced. The left lane is the alkaline hydrolysis ladder, and the right lane is RNase T1 digestion products. Marker sizes are labeled in yellow. The sizes of the cleavage products are
labeled in pink. Representative gel is from three independent experiments. (C) Structural superposition of the NTD-Cas14 (purpleblue and green, this study) and RNaseJ (pink, PDB ID: 4XWT).
(D) Detailed view of the catalytic pockets in the aligned structures of NTD-Cas14 and RNase J. Catalytic residues are shown as sticks, and zinc ions are represented as spheres. (E) Proposed
catalytic mechanism of Cas14 two-metal-ion catalysis. Two Zn2+ ions are depicted as gray circles, and the putatively involved water molecule is shown in blue. Hydrogen bonds and zinc
coordination are represented by dashed lines. Asp66, along with the water molecule, facilitates the nucleophilic attack of the scissile phosphate. His373 functions as a general acid to
protonate and stabilize the reaction products. (F) In vitro RNA cleavage assay showed that the zinc chelator (1,10-phenanthroline) prevents target cleavage, and this inhibition cannot be
reversed by the addition of Mg2+ or Mn2+. Representative gel is from three independent replicates. (G) Structural superposition of the NTD-Cas14 dimer (purpleblue and deepolive, this study)
and RNase J dimer (pink and cyan, PDB ID: 4XWT). (H) Size-exclusion chromatography analysis of NTD-Cas14 proteins in the presence of Mg2+, Mn2+, EDTA and 1,10-phenanthroline, respectively.
For gel source data, see Supplementary Fig. 1. EXTENDED DATA FIG. 7 BIOCHEMICAL ANALYSIS OF CAS14-MEDIATED TARGET RNA CLEAVAGE. (A) Schematic representation of the crRNA-target RNA duplex.
(B) Mapping of target RNA cleavage products by Cas14. Lane 1: RNase T1 hydrolysis ladder; Lane 2: 60-min cleavage products; Lane 3: 3′-Cy3 labeled 49-, 46-, 43-, 39-, 35-nt RNA oligos; Lane
4: 3-min cleavage products; Lane 5: 3′-Cy3 labeled 48- and 45-nt RNA oligos. The sizes of the RNA are indicated by numbers in yellow or pink. The gel is representative of three biological
replicates. For gel source data, see Supplementary Fig. 1. EXTENDED DATA FIG. 8 STRUCTURE OF THE INTERFERENCE COMPLEX IN POST STATES I AND II. (A-B) Cryo-EM structures (left panels) and
cartoon representations (middle panels) of Cas14-bound complexes at post-states I (A) and II (B). Right panels: Structural comparisons between post-state I and substrate-engaged state I (A),
or between post-state II and substrate-engaged state II (B). EXTENDED DATA FIG. 9 THE POTENTIAL ACTIVATION MECHANISM. (A) Superposition of substrate-engaged states I and II. Vector lengths
represent the movement of Cas14 domains. (B) Mutations of key residues that mediate the interactions between CTD-Cas14 and Cas7 failed to cleave the target RNA. The gel is representative of
three biological replicates. (C) Model of Cas14 binding and target RNA cleavage. Upon CTR binding, the Cas14 proteins interact with the CTR-bound Cas5–Cas7 complex in two states (I and II),
cleaving the CTR at two separate sites. When binding to NTR, the elongated Cas5–Cas7 filament enables the Cas14 proteins to bind at three different sites, resulting in the formation of three
major cleavage products (+I, I, and II). The Cas14 probably disassembles from the interference complex, and the Cas14-mediated cleavage products, such as the major cleavage I product, might
also serve as a substrate for subsequent cleavage rounds (dashed arrows). Unlike the type III system, the type VII system possesses an additional 3′-end repeat in its crRNA besides the
5′-end repeat. On the other hand, target RNA cleavage in the type VII system is affected by a complementary 5′-PFS but not the 3′-PFS, contrasting with the observations in the type III
system. (D) Structural superposition of the NTD-Cas14 (purpleblue and green, this study) with apo CPSF-73 (white, PDB ID: 2I7V) and target RNA-bound CPSF-73 (brown, PDB ID: 6V4X). Bottom:
The arrow indicates the conformational changes between apo CPSF-73 and target RNA-bound CPSF-73. For gel source data, see Supplementary Fig. 1. SUPPLEMENTARY INFORMATION SUPPLEMENTARY
INFORMATION Supplementary Notes 1 and 2 and Figs. 1–9. REPORTING SUMMARY SUPPLEMENTARY VIDEO 1 Recognition of cognate target RNA (CTR) by the Cas5–Cas7 complex and the subsequent recruitment
of Cas14 for target cleavage. Two substate-engaged states (I and II) are shown, and a zoomed-in view highlights the catalytic pocket located in the N-terminal region of the Cas14 protein.
SUPPLEMENTARY VIDEO 2 Recognition of non-cognate target RNA (NTR) by the Cas5–Cas7 complex and the subsequent recruitment of Cas14 for target cleavage. Three substate-engaged states (+I, I
and II) are shown, and a zoomed-in view highlights the catalytic pocket located in the N-terminal region of the Cas14 protein. SOURCE DATA SOURCE DATA EXTENDED DATA FIG. 1 RIGHTS AND
PERMISSIONS Springer Nature or its licensor (e.g. a society or other partner) holds exclusive rights to this article under a publishing agreement with the author(s) or other rightsholder(s);
author self-archiving of the accepted manuscript version of this article is solely governed by the terms of such publishing agreement and applicable law. Reprints and permissions ABOUT THIS
ARTICLE CITE THIS ARTICLE Yang, J., Li, X., He, Q. _et al._ Structural basis for the activity of the type VII CRISPR–Cas system. _Nature_ 633, 465–472 (2024).
https://doi.org/10.1038/s41586-024-07815-0 Download citation * Received: 19 March 2024 * Accepted: 11 July 2024 * Published: 14 August 2024 * Issue Date: 12 September 2024 * DOI:
https://doi.org/10.1038/s41586-024-07815-0 SHARE THIS ARTICLE Anyone you share the following link with will be able to read this content: Get shareable link Sorry, a shareable link is not
currently available for this article. Copy to clipboard Provided by the Springer Nature SharedIt content-sharing initiative