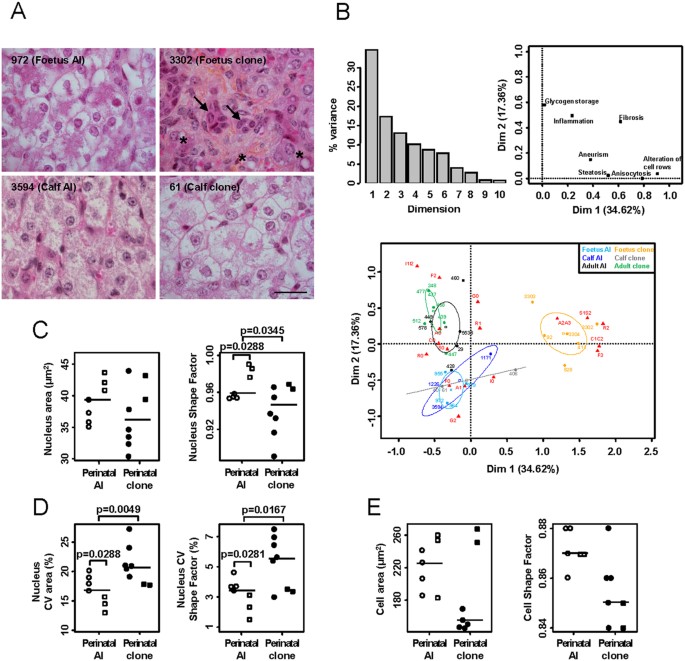
Altered dna methylation associated with an abnormal liver phenotype in a cattle model with a high incidence of perinatal pathologies
- Select a language for the TTS:
- UK English Female
- UK English Male
- US English Female
- US English Male
- Australian Female
- Australian Male
- Language selected: (auto detect) - EN
Play all audios:

ABSTRACT Cloning enables the generation of both clinically normal and pathological individuals from the same donor cells, and may therefore be a DNA sequence-independent driver of phenotypic
variability. We took advantage of cattle clones with identical genotypes but different developmental abilities to investigate the role of epigenetic factors in perinatal mortality, a
complex trait with increasing prevalence in dairy cattle. We studied livers from pathological clones dying during the perinatal period, clinically normal adult clones with the same genotypes
as perinatal clones and conventional age-matched controls. The livers from deceased perinatal clones displayed histological lesions, modifications to quantitative histomorphometric and
metabolic parameters such as glycogen storage and fatty acid composition, and an absence of birth-induced maturation. In a genome-wide epigenetic analysis, we identified DNA methylation
patterns underlying these phenotypic alterations and targeting genes relevant to liver metabolism, including the type 2 diabetes gene _TCF7L2_. The adult clones were devoid of major
phenotypic and epigenetic abnormalities in the liver, ruling out the effects of genotype on the phenotype observed. These results thus provide the first demonstration of a genome-wide
association between DNA methylation and perinatal mortality in cattle, and highlight epigenetics as a driving force for phenotypic variability in farmed animals. SIMILAR CONTENT BEING VIEWED
BY OTHERS COMPARATIVE ANALYSIS OF HISTONE H3K4ME3 MODIFICATIONS BETWEEN BLASTOCYSTS AND SOMATIC TISSUES IN CATTLE Article Open access 15 April 2021 EPIGENETIC CHANGES INDUCED BY IN UTERO
DIETARY CHALLENGE RESULT IN PHENOTYPIC VARIABILITY IN SUCCESSIVE GENERATIONS OF MICE Article Open access 05 May 2022 DEFECTIVE FOLATE METABOLISM CAUSES GERMLINE EPIGENETIC INSTABILITY AND
DISTINGUISHES _HIRA_ AS A PHENOTYPE INHERITANCE BIOMARKER Article Open access 17 June 2021 INTRODUCTION Although there is increasing evidence that epigenetics participates in phenotypic
variability in many species, epigenetic data underlying complex traits are scarce in livestock1, in part because epigenetic factors are confounded with the effects of DNA sequence
polymorphism2. One example of complex traits with increasing prevalence in dairy cattle is perinatal mortality3, which is an important issue for animal welfare, and is also detrimental to
both milk production and maternal health and fertility. Since perinatal mortality involves both genetic (breed of the dam, genetic merit of the sire) and non-genetic (herd management,
breeding method) factors, cloned animals with identical genotypes but different developmental abilities can be used to demonstrate the association between epigenetic factors and this trait.
The production of clones from an adult somatic cell is enabled by the capacity of the oocyte to reprogramme the epigenome of a differentiated nucleus to totipotency, and aberrant epigenetic
reprogramming is recognized as one of the leading causes of the developmental abnormalities encountered in clones4. Accordingly, DNA methylation defects have been reported in bovine clones,
at both the global level5,6 and at candidate loci7,8,9, but genome-wide views of the affected regions are still scarce10 and associations with phenotypic anomalies have not been clearly
demonstrated. Despite important advances in the procedures11,12,13,14, only 11.5% of cloned embryos transferred to recipient cows develop to term15. As well as the constant developmental
waste observed after implantation in cloned pregnancies16, the perinatal period is marked by important foetal and neonatal losses17,18,19. Foetal growth peaks towards the end of pregnancy
and makes considerable demands on the maternal nutrient supply. The impaired placental function observed in cloned pregnancies may hamper adequate exchanges between the dam and foetus,
resulting in these important perinatal losses and in metabolic disorders at the start of postnatal life20. Although the precise cause of death is unclear in most cases, large offspring
syndrome and large placenta, which are often associated with _hydrops fetalis_ and maternal hydrallantois, have frequently been observed in a context of clone stillbirth21. Episodic losses
are still reported up to six months of age, after which the health of clones is compatible with reproduction, lactation and meat production in most animals22,23,24,25. To date, adult clones
have been considered as being free of major abnomalities26, suggesting a selection of normal individuals through the gradual loss of pathological clones during development27. The large
number of abnormalities observed in perinatal clones, and the clinically normal phenotype of adult clones arising from the same donor cells, thus provide an unique opportunity to explore the
DNA sequence-independent mechanisms underlying perinatal mortality in cattle. Here we examined whether the phenotypic defects linked to perinatal mortality in clones could be associated
with altered DNA methylation profiles. The liver was selected for this study because it displays a grossly abnormal appearance (excessive size, enlarged lymphatic vessels, steatosis) at the
necropsy of deceased clones28,29, and is affected by deviations in the transcriptome30 and by DNA methylation defects at various developmental stages5,7. Furthermore, the liver plays a
fundamental role in the metabolic transition to postnatal life, through its ability to synthesize, store and export nutrients such as glucose and lipids31. This ability enables the
adaptation from a continuous supply of nutrients _in utero_ to enteral feeding after birth, which is precisely the stage through which many clones are unable to pass. We performed a
qualitative and quantitative analysis of the liver structure and composition in pathological perinatal clones (both foetuses and neonates), clinically normal adult clones with the same
genotypes as the perinatal deceased clones, and age-matched controls obtained by artificial insemination (AI). We report here on histomorphometric and metabolic parameters that were
specifically altered in the livers of pathological perinatal clones, and which might therefore represent markers of the hepatic pathologies encountered in cloned animals. Finally, we
identified DNA methylation patterns correlated to these phenotypic markers and targeting genes relevant to hepatic metabolism. Interestingly, these epigenetic patterns were similar to those
of adults, suggesting that an uncoupling of DNA methylation and age occurred in the pathological clones. RESULTS THE LIVERS OF PERINATAL CLONES DISPLAY PATHOLOGICAL FEATURES AND ALTERED
HISTOMORPHOMETRIC PARAMETERS Standard histological examination of the livers of clones and AI controls revealed marked lesions in some perinatal clones, such as fibrosis, generalized
anisocytosis (with some small hepatocytes and a few, large, vacuolated hepatocytes reflecting mild steatosis), and pseudo-canals of small hepatocytes. These lesions are illustrated Fig. 1A
in a representative individual (upper right panel). By contrast, some other perinatal animals displayed hepatocyte trabeculae of homogeneous size, with regular sized sinusoids and marked
intracytoplasmic glycogen storage appearing as white and granular matter (other panels). To identify groups of animals with similar histological features, we performed multiple
correspondence analysis (MCA)32 on scored lesions (Tables 1 and 2). Alterations to cell row organization, anisocytosis and, to a lesser extent, fibrosis and steatosis, contributed strongly
to dimension 1 which explained 35% of total variance (Fig. 1B, upper panels). Dimension 1 combined pathological features that appeared more frequently in the cloned foetuses, with the
frequent occurrence of categories R2 (no visible trabeculae), C1C2 (anisocytosis with some atypical cells), F3 (marked bridging fibrosis), S1S2 (moderate to important steatosis) and A2A3
(aneurysm) in these animals (lower panel). Dimension 2 combined histological features that highlighted the differences between other perinatal animals and adults with an important
contribution of glycogen storage, which was biased toward category G2 (diffuse glycogen storage) in perinatal controls and cloned calves. Although fibrosis (F2) and inflammation (I1I2) were
more frequently observed in adult clones than in adult AI, there was no significant difference between these two groups. These results therefore demonstrate that in the present cohort, all
clones that died before birth suffered from severe histological lesions of the liver. These findings caused us to determine quantitative measurements of these hepatic injuries by means of a
morphometric analysis. The nuclear area tended to be smaller in perinatal clones (Fig. 1C), and the nuclear shape was also altered, as revealed by the reduced shape factor. Consistent with
the anisocytosis observed in Fig. 1A,B, the nuclei of perinatal clones displayed greater heterogeneity in size and shape than those of perinatal AI controls (Fig. 1D; increased coefficients
of variation (CVs)). Perinatal clones tended to have smaller hepatocytes (p = 0.1145, Fig. 1E, left panel) with a more irregular shape (p = 0.0520, right panel) than AI controls. The
tendency towards smaller hepatocytes was more marked in cloned foetuses (which failed to accumulate glycogen) than in cloned calves, so we tested whether the cell size differed in
individuals with (G2) and without (G0) glycogen storage. The cell area was indeed significantly larger in the G2 group than in the G0 group which contained most cloned foetuses (median G2
group = 247 μm2; median G0 group = 153 μm2; p = 0.0007), showing that sizing the hepatocytes provided an indirect measure of the level of glycogen storage. No significant differences could
be found between adult clones and adult controls (Supplementary Fig. S1). Taken together, these data demonstrate that quantitative measures reflecting key histopathological features of
perinatal clones could be obtained by means of a morphometric analysis of hepatocytes. THE FATTY ACID COMPOSITION IS ALTERED IN PERINATAL CLONES To determine the molecular outcome of the
hepatosteatosis observed in some cloned foetuses, we then addressed the issue of whether the quantity and composition of fatty acids (FA) were modified in clones. Twenty types of long-chain
FAs were found in the perinatal animals, and 16 in the adults. Total FA levels of either membrane phospholipids or neutral lipids representing intracellular lipid storage, did not differ
significantly between the perinatal clones and perinatal controls, although the elevated FA content in neutral lipids confirmed marked steatosis in three clones (Fig. 2A). Principal
component analysis (PCA) run on the FA composition revealed that members of the omega 3 and omega 6 polyunsaturated FA families, such as docosahexaenoic acid (C22:6ω3) and arachidonic acid
(C20:4ω6), were major contributors to dimensions 1 and 2 which explained 58% of total variance (Fig. 2B, left and middle panels). Dimension 1 essentially discriminated adults from perinatal
clones and AI foetuses, while dimension 2 separated AI calves from the other animals (right panel). Cloned foetuses and cloned calves displayed an intermediary phenotype between AI foetuses
and AI calves. However, it is worth noting that the two oldest cloned calves, individuals 76 and 2263 (postnatal days 2 and 4), tended to be more similar to the AI calves (postnatal day 4).
Because the PCA highlighted C22:6ω3 and C20:4ω6 as potent FAs to partition the individuals according to age and clone status, we next focused on the C22:6ω3/C20:4ω6 ratio, which was
significantly elevated in perinatal clones, independently of the pre/postnatal stage, with respect to both phospholipids and neutral lipids (Fig. 2C). In addition to the C22:6ω3/C20:4ω6
ratio, several major differences were found between perinatal AI and perinatal clones in terms of FA composition (Supplementary Fig. S2). Minor differences were also detected between adult
clones and controls (Supplementary Fig. S3). Collectively, these data demonstrate that the FA composition was altered in the livers of the cloned animals. In perinatal clones, these
alterations particularly affected the bioactive polyunsaturated FAs C22:6ω3 and C20:4ω6. BIRTH-INDUCED LIVER MATURATION DOES NOT OCCUR IN PATHOLOGICAL PERINATAL CLONES To investigate whether
physiological maturation of the liver at birth had effects on the phenotypic parameters measured, we compared foetuses with calves in both the AI and clone groups. For most
histomorphometric parameters and FA features, we observed significant differences between the prenatal and postnatal stages in AI controls only (Figs 1C,D and 2A,C and Supplementary Fig.
S2). The absence of physiological changes in perinatal clones probably did not result solely from an uneven age distribution (i.e., most cloned calves analysed here were at pseudo-term
whereas the AI calves were at postnatal day 4). Indeed, the correlation between chronological age and phenotype was lost in perinatal clones regarding the morphometric parameters of
hepatocyte nuclei (except for the nucleus CV area; Fig. 3A and Supplementary Fig. S4). FA levels and composition also tended to correlate to chronological age in perinatal AI, with a
correlation coefficient of r ≥ 0.5 for most of the individual FAs examined (26 out of 34; Supplementary Fig. S4). By contrast, in perinatal clones, only six individual FAs tended to
correlate to chronological age. This tendency was exemplified by the total amounts of FAs and the percentage of omega 6 polyunsaturated FAs in phospholipids, both positively correlated to
chronological age, and the percentage of omega 6 polyunsaturated FAs in neutral lipids, which tended to correlate negatively to chronological age, all correlations being observed in AI
controls only (Fig. 3B). We concluded that birth-induced maturation did not occur and therefore that the biological age of the liver was altered in the pathological perinatal clones. TWO
DISTINCT SETS OF DIFFERENTIALLY METHYLATED REGIONS UNDERLIE THE NORMAL TRANSITION TO ADULT LIFE AND ABNORMAL LIVER PHYSIOLOGY The next objective was to investigate whether a correlation
could be established between the phenotypic modifications described above and altered DNA methylation profiles. We identified differentially methylated regions (DMRs) in gene promoters
displaying variations between perinatal animals and adults (246 age-related DMRs) and/or between clones and AI controls (83 cloning-related DMRs) (Supplementary Information). Compared to the
perinatal controls, adults were globally demethylated at age-related DMRs, indicating that dynamic changes in DNA methylation paralleled the normal transition from perinatal to adult life
(Supplementary Fig. S12). Similarly, pathological perinatal clones displayed aberrant hypomethylation at most age-related DMRs and cloning-related DMRs, suggesting epigenetic alterations
underlying an abnormal liver physiology. To highlight DMRs associated with phenotypic variations, we used multiple factor analysis (MFA)33. Three sets of quantitative variables were
integrated: methylation at age-related DMRs and cloning-related DMRs (“DMR” set, 282 variables), histomorphometric parameters measured on hepatocytes (“Morpho” set) and FA composition (“FA”
set). First of all, MFA was applied to the largest set of individuals with no missing data, which included adult controls, adult clones, AI foetuses and cloned foetuses. The first dimension
of MFA combined variables from the different sets that provided a clear partition between adults and foetuses (Fig. 4A, left panel). In the same way as for the FA and Morpho sets, the
coordinate of the “Group” illustrative variable on dimension 1 was close to 1, while its coordinate on dimension 2 was quite weak. This showed that partitioning as a function of groups was
particularly relevant on dimension 1, and that the phenotypic datasets made a major contribution to this (right panel). Variables in the three sets contributing to this partitioning could be
identified based on their strong correlation with dimension 1. Interestingly, the 182 DMRs significantly correlated to dimension 1 (listed in Supplementary Table S6) were almost exclusively
limited to age-related DMRs (Fig. 4B). The phenotypic variables most significantly correlated to dimension 1 are shown Fig. 4C and notably included modifications to the percentages of omega
3 and omega 6 polyunsaturated FAs. Consistent with the observation that only age-related DMRs contributed to dimension 1 of MFA, significant correlations were observed between these
phenotypic variables and the average methylation at age-related DMRs, but not at cloning-related DMRs (Fig. 4C). Gene ontology (GO) analysis of the 182 DMRs that correlated to dimension 1 of
MFA revealed an over-representation of genes involved in glycogen, lipid and cholesterol metabolism (Supplementary Fig. S14). This suggests that modifications to DNA methylation profiles
regulate the expression of genes involved in the major metabolic pathways of the liver during the switch towards an adult diet. Most of these genes were methylated in perinatal animals and
demethylated in adults, without there being any significant effect of cloning. Overall, these results show that when all the datasets were considered, the major source of variability that
could be identified was related to age and not to cloning. They also demonstrate that a substantial part of the age-related DMRs correlated to the phenotypic variations that distinguished
perinatal from adult animals. Dimension 2 of MFA tended to separate AI foetuses from cloned foetuses while adult clones and adult controls were not dissociable, suggesting that the second
important source of variability was related to the effects of cloning in foetuses only. To identify the variables that discriminated cloned and AI foetuses, we next ran MFA on a restricted
subset of foetuses in which a more detailed phenotypic characterization had been performed and the number of variables had been increased (three clones and four controls). Dimension 1
combined variables in the three sets that discriminated AI from clones, highlighting a cloned/AI origin as the principal source of variability between foetuses from both the phenotypic and
epigenetic points of view (Fig. 5A, left panel). The coordinate of the Group illustrative variable on dimension 1 was very close to 1, while its coordinate on dimension 2 was null,
demonstrating that only dimension 1 enabled partitioning as a function of the cloned/AI origin (Fig. 5A, right panel). As illustrated by their elevated coordinates on dimension 1, both the
phenotypic and DMR sets made a major contribution to this partitioning, while the FA set contributed equally to both dimensions 1 and 2. Dimension 2 therefore highlighted differences in FA
composition in some individuals, independently of their cloned/AI origin. Unlike the DMRs contributing to the separation according to age, the 148 DMRs that significantly correlated to
dimension 1 (listed in Supplementary Table S7) were evenly distributed among both age-related DMRs and cloning-related DMRs (Fig. 5B). The phenotypic variables that were significantly
correlated to dimension 1 reflected heterogeneity in the morphology of hepatocyte nuclei (CVs), glycogen storage (cell area), the shape of hepatocytes (cell shape factor), the balance
between omega 3 and omega 6 polyunsaturated FAs, and other FA features (Fig. 5C). Consistent with the MFA results, significant correlations with average methylation at both age-related DMRs
and cloning-related DMRs were confirmed for most of these phenotypic variables in a larger set of animals (eight to eleven animals, Fig. 5D). Taken together, these results highlighted the
phenotypic parameters that could distinguish pathological perinatal clones from perinatal AI controls and might therefore constitute markers of perinatal pathologies. They also demonstrated
that a subset of both age-related DMRs and cloning-related DMRs were correlated to these markers. GENES INVOLVED IN THE RESPONSE TO STRESS AND ENERGY METABOLISM ARE DEREGULATED IN
PATHOLOGICAL PERINATAL CLONES GO analysis of the 148 DMRs that significantly correlated to dimension 1 of MFA and contributed to the separation between perinatal clones and controls revealed
an over-representation of genes involved in the response to stress and stimuli (_NFKBIB, PPP5C, TCF7L2, COL1A1, IL6ST_) and in metabolic pathways (glycogen and steroids; Fig. 6A). As well
as _TCF7L2, G6PC3, PPP5C_ and _INSIG2_ listed in Fig. 6A, other key genes in energy metabolism such as _LDHA, SIRT2_ and _NDUFA4_ were epigenetically deregulated in perinatal clones. The
microarray data showed that these genes were hypomethylated in perinatal clones when compared to perinatal controls (Fig. 6B and Fig. 7A). The identification of _TCF7L2_ among the DMRs
correlated to dimension 1 of MFA was appealing, because this is a master gene for liver differentiation and function34,35. Furthermore, the DMR was analysed by pyrosequencing on an extended
cohort of animals (Fig. 7B), enabling a more detailed characterization of the relationships between methylation and phenotype. Among the phenotypic variables listed in Fig. 5C, five
correlated significantly to methylation at _TCF7L2_ DMR (Fig. 7C). Consistent with the function of _TCF7L2_ in the regulation of gluconeogenesis, the livers of cloned foetuses that failed to
store glycogen (reduced cell area) displayed a weaker methylation of _TCF7L2_ than those of cloned calves or controls with normal glycogen storage. Furthermore, _TCF7L2_ expression was
weaker in the livers of perinatal clones than in those of perinatal controls, and was positively correlated to methylation of the DMR (Fig. 7D). The gene start of bovine _TCF7L2,_ as defined
at the time of the microarray design and in the current versions of bovine genome annotation, maps on coding exons in other species. It is therefore likely that _TCF7L2_ DMR is centred on a
coding exon rather than on the promoter, which is consistent with a positive correlation between methylation and expression36. In conclusion, the methylation status of genes critical to
achieving a response to stress and to regulating energy metabolism was correlated to altered phenotypic parameters in pathological perinatal clones. For at least one of these genes,
methylation defects were associated with deregulated expression, which may have functional effects on the liver phenotype in terms of response to stress and energy metabolism. DISCUSSION
During this study, we explored the transition between late _in utero_ life, early postnatal life and adulthood in terms of functional and epigenetic adaptations of the liver, in both
conventional cattle and clones. Using multi-parameter correlation analyses, we demonstrated that normal physiological modifications associated with age, and phenotypic alterations related to
perinatal mortality, were both underlain by specific changes to DNA methylation patterns. In agreement with previous studies28,29, we observed important histological lesions in pathological
cloned foetuses which included steatosis and severe fibrosis. In addition, we found that the fibrotic livers contained pseudo-canals of small hepatocytes reminiscent of regenerative nodules
in cirrhotic livers. Unlike the foetal clones, the adult clones and cloned calves were not dissociable from age-matching AI controls in an analysis performed on scored lesions, suggesting
that the liver damage affecting perinatal clones might be milder if gestation could be maintained until term. However, major alterations to FA composition and an absence of birth-induced
maturation were indicative of hepatic defects in cloned calves as well. Modifications to quantitative histological parameters, such as the nuclear alterations observed in pathological
perinatal clones, offered further evidence that they suffered from abnormal liver physiology and metabolism. Indeed, the size and shape of the interphase nucleus are closely dependent on
cell size, function and metabolism and can be modified by ageing, oxidative stress and pathology37,38,39. Steatosis may also have contributed to the nuclear alterations we observed, since
lipid vesicles compress the nucleus at the periphery of the hepatocyte, leading to its deformation. A Japanese survey of the necropsy findings relative to clones at various postnatal stages
reported hepatic lesions in 14 out of 38 neonatal deaths, whereas this number dropped to two out of 25 at later stages of life27. This survey, together with the present study, both support
the idea that pathological clones are characterized by an improper reprogramming of liver functions, which impairs their ability to adapt to postnatal life. We found epigenetic alterations
correlated to phenotypic anomalies in genes related to the mitochondrial function (_NDUFA4, LDHA_ and _SIRT2_), pointing towards mitochondrial defects as a potential origin of the liver
damage seen in the pathological perinatal clones. In the context of chronic hepatitis C and non-alcoholic fatty liver disease (NAFLD), mitochondrial defects, together with inflammation and
oxidative stress, are indeed suspected of causing the progression of hepatosteatosis towards more severe liver injury40,41. Interestingly, the hypothesis of mitochondrial defects in clones
has been supported by other studies42. Variations in the proteomic profile of liver mitochondria have been observed in deceased newborn bovine clones43 and an altered expression of genes
involved in oxidative phosphorylation, including _NDUFA4,_ has been reported in the lungs of deceased cloned piglets44. _LDHA_ is involved in the final step of glycolysis and is up-regulated
in senescent cells with mitochondrial dysfunction45, and _SIRT2_ is a key gene controlling entry into the pentose phosphate pathway46. The metabolic transition from oxidative
phosphorylation to the glycolysis/pentose phosphate pathway favours NADPH-dependent antioxidant responses over energy expenditure, and occurs in the event of impaired mitochondrial function,
ageing and cancer47. A genetic deficiency leading to an unbalanced metabolite flux in the pentose phosphate pathway results in an accumulation of reactive oxygen species, steatosis and
fibrosis/cirrhosis in the livers of mice, and to _hydrops fetalis_ and liver cirrhosis in human infants48. It is therefore possible that the similar symptoms induced by cloning may be caused
by inadequate energy partitioning between the pentose phosphate pathway and oxidative metabolism. Through its storage, export and β-oxidation of lipids, and lipogenesis, the liver plays a
central role in lipid and FA metabolism in both pre-ruminant and ruminant cattle49, and several studies have reported changes to this metabolism in bovine clones23,50,51,52. At the
blastocyst stage, different lipid metabolites were detected in the culture medium of two types of cloned embryos with contrasting term development abilities50. A transcriptomic analysis of
cloned elongated conceptus highlighted the deregulation of genes involved in lipid and FA metabolism51, and in adult clones, both milk and meat displayed slight differences in their FA
composition when compared to controls bred in the same facility23,52. The present study confirmed the small deviation in FA metabolism in an independent cohort of adult clones, and in
another tissue, the liver. It also revealed important changes to FA metabolism in pathological perinatal clones, with an elevation of the C22:6ω3/C20:4ω6 ratio (which is related to the
balance between anti- and pro-inflammatory FA derivatives) in both membrane and intracellular lipid stores. Given the lesions observed in the pathological perinatal clones, this result was
surprising, since many studies have reported the beneficial actions of dietary omega 3 polyunsaturated FAs, including C22:6ω3, on hepatosteatosis and fibrosis (reviewed in ref. 53). The
elevated C22:6ω3/C20:4ω6 ratio observed in pathological perinatal clones may have reflected a response to the hepatic injuries by boosting the pool of anti-inflammatory precursors such as
C22:6ω3. Otherwise, it may have indicated the effects of a higher C20:4ω6 consumption in order to generate pro-inflammatory metabolites contributing to disease. During this study, we
identified two potential candidates with functions in polyunsaturated FA metabolism that displayed epigenetic alterations in pathological perinatal clones: _ALOX15_, which metabolises
C20:4ω6 to promote liver damage in hypercholesterolaemic mice54, and _CYB5R2 (LOC536960_ in Supplementary Tables S4 and S7), which is involved in the desaturation and elongation of FAs and
is a target of myristoylation55. Interestingly, myristic acid (C14:0) levels were raised in the membranes of our perinatal clones, and the protein encoded by _SIRT2_ (which was
differentially methylated in the perinatal clones) has been reported as displaying potent demyristoylase activity56. The regulation of _CYB5R2_ at the gene level by methylation, and at the
post-translational level by myristoylation, may therefore participate in the peculiar metabolism of omega 3 polyunsaturated FAs in pathological perinatal clones. Hepatosteatosis and an
absence of glycogen storage in cloned foetuses suggested a switch of intracellular energy stores from carbohydrates to lipids. We found aberrant DNA methylation profiles in two major
effectors of the Wnt signalling pathway (_DVL2_ and _TCF7L2_) that may be related to the impaired carbohydrate metabolism in perinatal clones. The functions of Wnt signalling in regulating
hepatic glucose metabolism have been reported elsewhere57, and in humans, genetic variants and aberrant methylation profiles of _TCF7L2_ have been associated with the development of type 2
diabetes58,59, increased NAFLD and fibrosis60. In line with a report showing that _TCF7L2_ is necessary for gluconeogenesis and glycogen accumulation in the livers of neonates34, we
demonstrated that _TCF7L2_ methylation was positively correlated to both _TCF7L2_ expression and hepatic glycogen storage in the perinatal clones. By contrast, others have shown that
_TCF7L2_ is a negative regulator of gluconeogenesis in adults61. These antagonistic roles may be a consequence of the complex transcriptional network in which _TCF7L2_ is involved, with both
transcriptional repressor and activator functions of its product depending on the interacting partners62. Because of this central position in the hepatic transcriptional network, _TCF7L2_
is a master gene of liver phenotype, not only for glucose metabolism but also for that of lipids and amino acids35. The altered expression we observed in perinatal clones may therefore drive
major pathophysiological changes in the liver, leading to NAFLD-related symptoms. In the perinatal clones, we also found epigenetic alterations in _G6PC3,_ a target of _TCF7L2_35,62 which
encodes a rate-limiting enzyme in gluconeogenesis. Together with other gluconeogenic genes, _G6PC3_ is induced near term in response to the glucocorticoid surge, allowing glycogen to
accumulate in order to supply energy to the newborn calf during the first hours post-partum31. This process failed to occur in our pathological cloned foetuses, but was at least partially
corrected in the pathological cloned calves that were treated with dexamethasone before caesarean section (C-section). Dexamethasone, in combination with pre- or postnatal ACTH
administration, is able to restore glycogen storage in the livers of premature calves63. It is therefore possible that in response to exogenous dexamethasone, the cloned calves initiated
endogenous glucose production independently of the epigenetic deregulation of _G6PC3_. The absence of glycogen storage, and the altered correlation between chronological age and the
phenotype of the liver, indicated impaired liver maturation in the pathological perinatal clones. We demonstrated that as the normal transition to postnatal life occurred, hepatocytes became
larger and acquired more regular and larger nuclei; the whole parenchyma matured towards a more homogeneous organization, and the membranes were enriched in FAs (and particularly in omega 6
polyunsaturated FAs that in parallel were depleted from intracellular FA stores). In pathological perinatal clones, all these processes were affected to different degrees, showing that the
administration of dexamethasone was not sufficient to prevent a failure to mature towards a functional liver. In particular, the absence of maturation in terms of FA composition might have
important consequences on membrane fluidity64 and on the FA composition of triglycerides exported by the liver. Cloned calves displayed normal cortisol secretion in response to ACTH65,
suggesting that corticoid signalling, rather that corticoid secretion, was altered in perinatal clones. Altered corticoid signalling in perinatal clones may be related to the epigenetic
deregulation of _PPP5C,_ which antagonizes the anti-lipogenic actions of glucocorticoid receptor66. One can speculate that deregulated levels of _PPP5C_ in the livers of cloned foetuses
might impair the activation of anti-lipogenic genes in response to the glucocorticoid surge, leading to the derivation of gluconeogenic substrates toward fat accumulation. The inability of
the immature liver to produce endogenous glucose may result in an increased dependence on maternal glucose, a hypothesis supported by reduced maternal plasma glucose levels and an elevated
expression of placental glucose transporters in late cloned pregnancies67. To our knowledge, this study is the first demonstration of an association between DNA methylation and liver
phenotypic variations in cattle at a genome-wide scale. We showed that epigenetic alterations affected the promoters of genes relevant to hepatic metabolism in pathological perinatal clones.
Using multi-parameter correlation analyses, we also revealed an association between these epigenetic alterations and phenotypic defects related to perinatal mortality. We did not observe
any major physiological and epigenetic abnormalities in the livers of adult clones arising from the same donor cells as the perinatal clones, ruling out the effects of genotype on the
phenotype observed. Our data therefore highlight epigenetics as a driving force behind phenotypic variability in farmed animals, and support the view that better control of the conditions of
development is required in order to reduce perinatal losses and improve the long-term health of livestock species. METHODS ANIMALS AND THE COLLECTION OF LIVER SAMPLES All the methods
described here were implemented in accordance with EU guidelines and regulations (directive 2010/63/UE), and all the experimental protocols were approved by the INRA local Ethics Committee
(COMETHEA, authorization number 12/160). _Post-mortem_ liver samples were obtained from 36 Holstein cattle: eleven perinatal clones of two distinct genotypes displaying pathological lesions,
nine adult clones (mostly clinically normal) of four distinct genotypes including the same genotypes as the perinatal clones, seven healthy perinatal controls resulting from AI and nine
healthy adult AI controls. The animals, and the experiments performed on the liver samples, are described in Table 1. Because postnatal life induces considerable maturation of the liver, for
the phenotypic investigations we considered four groups in perinatal animals: foetus AI, foetus clones, calf AI and calf clones. Animals that were the most homogeneous in terms of age,
breeding and production period were used for the genome-wide methylation analysis and constituted the so-called “microarray cohort” (Supplementary Information). The “extended cohort”, used
for pyrosequencing and phenotypic characterization, was obtained by including more animals in the microarray cohort, essentially perinatal clones and AI calves. Livers were sampled during
the necropsy examination and were fixed immediately in 10% neutral buffered formalin or snap-frozen in liquid nitrogen and stored at −80 °C until use. Due to sampling limitations, autolysis
or RNA degradation, not all the animals could be used for all the analyses. This cohort of clones represented a decade of cloning during which the same procedure was applied continuously.
For both clinically normal adult clones and pathological perinatal clones, somatic cell nuclear transfer was performed as described elsewhere12,65, using ear skin fibroblasts from four adult
Holstein females. Bovine ovaries were collected from a slaughterhouse and cumulus oocyte complexes were aspirated from follicles 2–7 mm in diameter. Recipient oocytes were matured _in
vitro_, and the metaphase II and polar body chromatin were removed 20–22 h post-maturation. For embryo reconstitution, an isolated donor cell was inserted under the _zona pellucida_ of the
enucleated oocyte and fused by electrostimulation. Embryos were seeded with Vero cells and cultured in B2 medium (CCD) supplemented with 2.5% foetal calf serum (Life Technologies). By day 7,
blastocysts had been transferred into synchronized recipient heifers (two blastocysts per heifer), and the pregnant recipients were monitored by ultrasound imaging as recommended by the
International Embryo Transfer Society (www.iets.org/pdf/hasac-healthassessmentcare.pdf). From gestational days 257 to 273, recipients positively diagnosed with hydrallantois were slaughtered
at the INRA experimental facilities or delivered by C-section whenever possible, and the foetuses were processed for necropsy. Gestations were pursued until pseudo-term (gestational days
276 to 282) in the remaining recipients that were negative for hydrallantois, which represented half of the total recipients still pregnant at gestational day 150. To induce foetal
maturation, a single dose of dexamethasone (20 mg) was injected 24 h before the C-section, and neonatal care was ensured in compliance with the guidelines of the International Embryo
Transfer Society. Clones that died spontaneously within 4 days of birth were processed for necropsy, alongside those slaughtered because of severe limb malformations. Clones with a
detectable heartbeat at birth were considered to be calves even if they died shortly after. All the other clones used here grew to adulthood and had a normal reproductive history, although
their ages at culling tended to be younger than the controls (median ages at culling: 5 years for clones and 6.5 years for AI controls). Perinatal and adult AI controls were maintained at
the same experimental farm as the clones (UCEA, INRA, France), and both clones and controls were fed the same diet after birth. Adults were essentially fed with fodder and silage, depending
on the physiological lactation stage. The recipients and mothers of all perinatal animals belonging to the microarray cohort were fed exclusively with fodder. In an attempt to improve the
condition of the recipients of clones and thereby increase the chances of obtaining full-term gestations, other perinatal animals (individuals 40, 61, 76, 92, 1171, 1226 and 3594) were
exposed to a different diet during gestation (fodder plus silage). HISTOLOGICAL EVALUATIONS Samples were embedded in paraffin wax and 6 μm-thick sections were routinely stained with
haemalin-eosin-saffron. For 30 animals, seven types of lesions or histological features were scored by a skilled pathologist (Tables 1 and 2). Morphometric measurements were performed using
a digital camera (Nikon DXM 1200) combined with Nikon Imaging Software (Nikon). Microscopic fields were selected randomly on haemalin-eosin-saffron-stained sections using high magnification.
Nuclear size and shape were used as indicators of the anisocytosis observed in some individuals. For at least 200 hepatocyte nuclei per animal on several separate fields, minimum and
maximum Feret diameters, total area, perimeter and shape factor (ranging from 0 to 1, with 1 corresponding to perfect circles) were measured. As the size parameters were highly correlated,
only the area and shape factor were considered. Furthermore, hepatocyte size and shape were used to determine available energy stores. For perinatal animals in which the cell membranes of
hepatocytes were clearly observable, cell total area and shape factor were measured on 35 hepatocytes on several separate fields. For each animal and histomorphometric parameter, the mean
and CV were calculated. AI controls and clones were compared using a permutation test for two independent samples (Monte-Carlo sampling of 100,000 permutations), which is a non-parametric
test suited to small samples. For perinatal animals, a stratified permutation test was used to take account of the stage (pre/postnatal). Foetuses and calves were then compared independently
within each group. Correlations related to morphometric measurements were estimated using Spearman’s rank correlation test. FATTY ACID COMPOSITION Lipids corresponding to 100 mg of hepatic
tissue were extracted using chloroform-methanol. Phospholipids were separated from non-phosphorous lipids on silica acid cartridges. The phospholipids and neutral lipids were transmethylated
with 7% Boron trifluoride methanol (Sigma-Aldrich). The methyl esters of phospholipids or neutral FAs were analysed by gas chromatography coupled to FID (Gas Chromatograph 3900 Varian) on
an Econo-Cap EC-WAX capillary column, using heptadecanoic acid (C17:0) as the internal standard68. The results were expressed as mg/g liver or as percentages of total FAs. FAs that were only
detected as traces were not considered. Statistical analyses relative to FA composition were performed as explained for the histological evaluation. EPIGENETIC ANALYSES The detailed methods
are described in the Supplementary Information. Briefly, a Roche-NimbleGen 3 × 720 K microarray targeting the upstream region (−2000 to +1360 bp relative to the gene start) of 21,296 bovine
genes was designed. Half of these upstream regions contained a CpG island. DNA extraction from the liver samples, methylated DNA immunoprecipitation (MeDIP) and quality controls were
performed as described elsewhere69. To prevent any technical biases, the products of 9–10 independent MeDIP experiments were pooled for each animal. After moderate genome amplification, the
pooled MeDIP reactions and corresponding input DNA were labelled with Cy3 and Cy5 and hybridized on the microarray, with technical dye-swaps for every sample. Probes with signal enrichment
in the MeDIP sample (“enriched probes”) were identified and an anchor-extension strategy was used to delineate 15,885 clusters of probes enriched under at least one condition (regions of
interest). 246 age-related DMRs and 83 cloning-related DMRs were identified using an R package designed to analyse point patterns, which was therefore appropriate for binary data (enriched
_vs._ not enriched). Three models were built: one full model taking account of both age and cloning (i) and two alternative models taking account of age only (ii) or cloning only (iii). The
interaction between age and cloning was not considered. Indeed, a more robust estimation of only two parameters was preferred, given the limited sample size (n = 26). To identify
cloning-related DMRs and age-related DMRs, the full model was compared with alternative models (ii) and (iii), respectively. All 15,885 regions were tested and the resulting p-values were
corrected for multiple testing. A given region was considered as a cloning-related DMR if the full model fitted the observations significantly better than alternative model (ii) (adjusted
p-value < 0.05). Similarly, a given region was considered as an age-related DMR if the full model fitted the observations significantly better than alternative model (iii). The microarray
results were validated by the pyrosequencing analysis of 12 DMRs (115 analysed CpGs) on the extended cohort (n = 35). MULTIVARIATE ANALYSES Some theoretical background on the multivariate
analyses used (MCA, PCA and MFA) is provided in the Supplementary Methods. All multivariate analyses were computed using the FactoMineR R package. MCA (which has proved its usefulness in the
field of histopathology32) was run on the set of qualitative data generated by scoring seven types of lesions in 30 animals (Tables 1 and 2). To prevent any distortion of the dimensions due
to poorly represented categories, those containing fewer than four individuals (G1, S1, S2, A3, and I2) were merged together or with adjacent categories, generating the intermediate
categories G1G2, S1S2, A2A3, and I1I2. PCA was computed on 22 quantitative variables representing percentages of individual FAs measured in 34 individuals and detected in both adults and
perinatal animals (C14:0, C16:0, C16:1ω7, C18:0, C18:1ω9, C18:2ω6, C18:3ω3, C20:3ω6, C20:4ω6, C20:5ω3, C22:4ω6, C22:5ω3, C22:6ω3 in both phospholipids and neutral lipids). Rank-converted
percentages were used to homogenize the distributions. In the results presented in Fig. 2B, the PL C18:1ω9, NL C20:3ω6, PL C22:4ω6 and NL C20:5ω3 variables, which were respectively highly
correlated to NL C18:1ω9, NL C20:4ω6, PL C20:4ω6, NL C22:5ω3, were removed, but this did not substantially change the results. MFA, which enables an estimation of the relationships between
sets of variables measured in the same individuals without _a priori_ modelling33, was run on three sets of quantitative variables: the “DMR” set, the “Morpho” set and the “FA” set. The DMR
set included all age-related DMRs and cloning-related DMRs (282 in total); the variables considered were the percentage of enriched probes , where Eri is the number of enriched probes for
DMR r and individual i, and Tr is the number of probes included in DMR r. The variables included in the Morpho and FA sets were as follows: Fig. 4A shows the Morpho set containing four
variables (mean area and mean shape factor measured on hepatocyte nuclei and the corresponding CVs) and the FA set including rank-converted percentages or ratios of FAs detected in both
adult and perinatal animals: C14:0, C16:0, C16:1ω7, C18:0, C18:1ω9, C18:2ω6, C18:3ω3, C20:3ω6, C20:4ω6, C20:5ω3, C22:4ω6, C22:5ω3, C22:6ω3, total saturated FAs, total monounsaturated FAs,
total polyunsaturated FAs, omega 6 polyunsaturated FAs, omega 3 polyunsaturated FAs, omega 3/omega 6, polyunsaturated FAs/saturated FAs, saturated FAs/unsaturated FAs, monounsaturated
FAs/saturated FAs, C18:2ω6/C20:4ω6 and C22:6ω3/C20:4ω6 for both phospholipids and neutral lipids (48 variables). Additional variables were considered in Fig. 5A: mean cell area and mean cell
shape factor measured on hepatocytes and the corresponding CVs (total of eight variables for the Morpho set), and PL 18:3ω6, PL 20:0, NL C14:1ω9, NL C15:0, NL C15:1ω9, NL C16:1ω9, NL
C18:3ω6 and NLC18:1ω7 (total of 56 variables for the FA set). MFA was computed on these three datasets, with the animal group set as the illustrative variable. Individuals that constituted
missing data for the considered variables were excluded from the analysis. In Figs 4C and 5C, correlations between the most contributory Morpho and FA variables and average values for
methylation at age-related DMRs or cloning-related DMRs were confirmed using Spearman’s rank correlation test on an extended panel of animals. The variables considered for average
methylation at age-related DMRs and cloning-related DMRs were Piage and Picloning, respectively, where and n is the total number of DMRs (n = 246 for age-related DMRs and n = 83 for
cloning-related DMRs). GO analysis of the DMRs correlated to dimension 1 of MFA was performed using the PANTHER database70. QUANTITATIVE PCR RNA was extracted with Trizol reagent
(Invitrogen) according to the manufacturer’s instructions, and reverse transcription and real-time quantitative PCR were performed as described elsewhere71. GeNorm software72 was used to
select _RPLP0, RPL19_ and _YHAWZ_ as reference genes for normalization and to obtain normalization factors. The primers for _RPLP0_ and _RPL19_ are described elsewhere71, as are the primers
for _YHAWZ_73. The primers for _TCF7L2_ were as follows (5′-3′): bTCF7L2_ex5_F1, AGCTGACGTAGACCCCAAAA; bTCF7L2_ex6_R1, TAGCGGATGGGGGATTTGTC. The results were presented as the mean relative
expression calculated for the sample in four replicates. Statistical analyses were performed as described in the section on histological evaluation. ADDITIONAL INFORMATION ACCESSION CODES:
The MeDIP-chip dataset supporting the results of this article is available in the NCBI Gene Expression Omnibus database (http://www.ncbi.nlm.nih. gov/geo/) under accession number GSE73028.
HOW TO CITE THIS ARTICLE: Kiefer, H. _et al_. Altered DNA methylation associated with an abnormal liver phenotype in a cattle model with a high incidence of perinatal pathologies. _Sci.
Rep._ 6, 38869; doi: 10.1038/srep38869 (2016). PUBLISHER'S NOTE: Springer Nature remains neutral with regard to jurisdictional claims in published maps and institutional affiliations.
REFERENCES * Ibeagha-Awemu, E. M. & Zhao, X. Epigenetic marks: regulators of livestock phenotypes and conceivable sources of missing variation in livestock improvement programs.
Frontiers in genetics 6, 302, doi: 10.3389/fgene.2015.00302 (2015). Article CAS PubMed PubMed Central Google Scholar * Goddard, M. E. & Whitelaw, E. The use of epigenetic phenomena
for the improvement of sheep and cattle. Frontiers in genetics 5, 247, doi: 10.3389/fgene.2014.00247 (2014). Article CAS PubMed PubMed Central Google Scholar * Mee, J. F.,
Sanchez-Miguel, C. & Doherty, M. Influence of modifiable risk factors on the incidence of stillbirth/perinatal mortality in dairy cattle. Veterinary journal 199, 19–23, doi:
10.1016/j.tvjl.2013.08.004 (2014). Article PubMed Google Scholar * Niemann, H. Epigenetic reprogramming in mammalian species after SCNT-based cloning. Theriogenology 86, 80–90, doi:
10.1016/j.theriogenology.2016.04.021 (2016). Article CAS PubMed Google Scholar * Hiendleder, S. et al. Tissue-specific effects of _in vitro_ fertilization procedures on genomic cytosine
methylation levels in overgrown and normal sized bovine fetuses. Biology of reproduction 75, 17–23, doi: 10.1095/biolreprod.105.043919 (2006). Article CAS PubMed Google Scholar * de
Montera, B. et al. Quantification of leukocyte genomic 5-methylcytosine levels reveals epigenetic plasticity in healthy adult cloned cattle. Cellular reprogramming 12, 175–181, doi:
10.1089/cell.2009.0062 (2010). Article CAS PubMed PubMed Central Google Scholar * Couldrey, C. & Lee, R. S. DNA methylation patterns in tissues from mid-gestation bovine foetuses
produced by somatic cell nuclear transfer show subtle abnormalities in nuclear reprogramming. BMC developmental biology 10, 27, doi: 10.1186/1471-213X-10-27 (2010). Article CAS PubMed
PubMed Central Google Scholar * Smith, L. C., Therrien, J., Filion, F., Bressan, F. & Meirelles, F. V. Epigenetic consequences of artificial reproductive technologies to the bovine
imprinted genes SNRPN, H19/IGF2, and IGF2R. Frontiers in genetics 6, 58, doi: 10.3389/fgene.2015.00058 (2015). Article CAS PubMed PubMed Central Google Scholar * Zhang, S. et al.
Aberrant DNA methylation reprogramming in bovine SCNT preimplantation embryos. Scientific reports 6, 30345, doi: 10.1038/srep30345 (2016). Article ADS CAS PubMed PubMed Central Google
Scholar * Su, J., Wang, Y., Xing, X., Liu, J. & Zhang, Y. Genome-wide analysis of DNA methylation in bovine placentas. BMC genomics 15, 12, doi: 10.1186/1471-2164-15-12 (2014). Article
PubMed PubMed Central Google Scholar * Kato, Y. et al. Eight calves cloned from somatic cells of a single adult. Science 282, 2095–2098 (1998). Article ADS CAS PubMed Google Scholar
* Vignon, X. et al. Developmental potential of bovine embryos reconstructed from enucleated matured oocytes fused with cultured somatic cells. _Comptes rendus de l’Academie des sciences_.
Serie III, Sciences de la vie 321, 735–745 (1998). CAS Google Scholar * Oback, B. & Wells, D. N. Cloning cattle: the methods in the madness. Advances in experimental medicine and
biology 591, 30–57, doi: 10.1007/978-0-387-37754-4_3 (2007). Article PubMed Google Scholar * Ross, P. J. & Cibelli, J. B. Bovine somatic cell nuclear transfer. Methods in molecular
biology 636, 155–177, doi: 10.1007/978-1-60761-691-7_10 (2010). Article PubMed Google Scholar * Keefer, C. L. Artificial cloning of domestic animals. Proceedings of the National Academy
of Sciences of the United States of America 112, 8874–8878, doi: 10.1073/pnas.1501718112 (2015). Article ADS CAS PubMed PubMed Central Google Scholar * Heyman, Y. Nuclear transfer: a
new tool for reproductive biotechnology in cattle. Reproduction, nutrition, development 45, 353–361, doi: 10.1051/rnd:2005026 (2005). Article PubMed Google Scholar * Batchelder, C. A. et
al. Perinatal physiology in cloned and normal calves: hematologic and biochemical profiles. Cloning and stem cells 9, 83–96, doi: 10.1089/clo.2006.0038 (2007). Article CAS PubMed Google
Scholar * Batchelder, C. A. et al. Perinatal physiology in cloned and normal calves: physical and clinical characteristics. Cloning and stem cells 9, 63–82, doi: 10.1089/clo.2006.0037
(2007). Article CAS PubMed Google Scholar * Hill, J. R. Incidence of abnormal offspring from cloning and other assisted reproductive technologies. Annual review of animal biosciences 2,
307–321, doi: 10.1146/annurev-animal-022513-114109 (2014). Article PubMed Google Scholar * Chavatte-Palmer, P. et al. Review: Placental perturbations induce the developmental
abnormalities often observed in bovine somatic cell nuclear transfer. Placenta 33 Suppl, S99–S104, doi: 10.1016/j.placenta.2011.09.012 (2012). Article CAS PubMed Google Scholar *
Constant, F. et al. Large offspring or large placenta syndrome? Morphometric analysis of late gestation bovine placentomes from somatic nuclear transfer pregnancies complicated by
hydrallantois. Biology of reproduction 75, 122–130, doi: 10.1095/biolreprod.106.051581 (2006). Article CAS PubMed Google Scholar * Tian, X. C. et al. Meat and milk compositions of bovine
clones. Proceedings of the National Academy of Sciences of the United States of America 102, 6261–6266, doi: 10.1073/pnas.0500140102 (2005). Article ADS CAS PubMed PubMed Central
Google Scholar * Heyman, Y. et al. Assessing the quality of products from cloned cattle: an integrative approach. Theriogenology 67, 134–141, doi: 10.1016/j.theriogenology.2006.09.020
(2007). Article CAS PubMed Google Scholar * Watanabe, S. Somatic cell cloned cattle and their progeny produced in Japan: a report for animal health and characteristics of animal
products. Vol. 12 (National Institute of Livestock and Grassland Science, NARO, 2011). * Polejaeva, I. A. et al. Longitudinal study of reproductive performance of female cattle produced by
somatic cell nuclear transfer. PloS one 8, e84283, doi: 10.1371/journal.pone.0084283 (2013). Article ADS CAS PubMed PubMed Central Google Scholar * Sinclair, K. D. et al. Healthy
ageing of cloned sheep. Nature communications 7, 12359, doi: 10.1038/ncomms12359 (2016). Article ADS CAS PubMed PubMed Central Google Scholar * Watanabe, S. Effect of calf death loss
on cloned cattle herd derived from somatic cell nuclear transfer: clones with congenital defects would be removed by the death loss. Animal science journal = Nihon chikusan Gakkaiho 84,
631–638, doi: 10.1111/asj.12087 (2013). Article PubMed Google Scholar * Chavatte-Palmer, P. et al. Health status of cloned cattle at different ages. Cloning and stem cells 6, 94–100, doi:
10.1089/1536230041372274 (2004). Article CAS PubMed Google Scholar * Maiorka, P. C. et al. Vascular alterations underlie developmental problems manifested in cloned cattle before or
after birth. PloS one 10, e0106663, doi: 10.1371/journal.pone.0106663 (2015). Article CAS PubMed PubMed Central Google Scholar * Herath, C. B. et al. Developmental aberrations of liver
gene expression in bovine fetuses derived from somatic cell nuclear transplantation. Cloning and stem cells 8, 79–95, doi: 10.1089/clo.2006.8.79 (2006). Article CAS PubMed Google Scholar
* Hammon, H. M., Steinhoff-Wagner, J., Schonhusen, U., Metges, C. C. & Blum, J. W. Energy metabolism in the newborn farm animal with emphasis on the calf: endocrine changes and
responses to milk-born and systemic hormones. Domestic animal endocrinology 43, 171–185, doi: 10.1016/j.domaniend.2012.02.005 (2012). Article CAS PubMed Google Scholar * Meyer, N.,
Ferlicot, S., Vieillefond, A., Peyromaure, M. & Vielh, P. [Contribution of multiple correspondence analysis in histopathology]. Annales de pathologie 24, 149–160 (2004). Article PubMed
Google Scholar * Escofier, B. & Pagès, J. Multiple factor analysis (AFMULT package). Computational Statistics & Data Analysis 18, 121–140 (1994). Article MATH Google Scholar *
Boj, S. F. et al. Diabetes risk gene and Wnt effector Tcf7l2/TCF4 controls hepatic response to perinatal and adult metabolic demand. Cell 151, 1595–1607, doi: 10.1016/j.cell.2012.10.053
(2012). Article CAS PubMed Google Scholar * Norton, L. et al. The mechanisms of genome-wide target gene regulation by TCF7L2 in liver cells. Nucleic acids research 42, 13646–13661, doi:
10.1093/nar/gku1225 (2014). Article CAS PubMed PubMed Central Google Scholar * Schubeler, D. Function and information content of DNA methylation. Nature 517, 321–326, doi:
10.1038/nature14192 (2015). Article ADS CAS PubMed Google Scholar * Webster, M., Witkin, K. L. & Cohen-Fix, O. Sizing up the nucleus: nuclear shape, size and nuclear-envelope
assembly. Journal of cell science 122, 1477–1486, doi: 10.1242/jcs.037333 (2009). Article CAS PubMed PubMed Central Google Scholar * Barascu, A. et al. Oxydative stress alters nuclear
shape through lamins dysregulation: a route to senescence. Nucleus 3, 411–417, doi: 10.4161/nucl.21674 (2012). Article PubMed PubMed Central Google Scholar * Edens, L. J., White, K. H.,
Jevtic, P., Li, X. & Levy, D. L. Nuclear size regulation: from single cells to development and disease. Trends in cell biology 23, 151–159, doi: 10.1016/j.tcb.2012.11.004 (2013). Article
CAS PubMed Google Scholar * Kitase, A. et al. _In situ_ detection of oxidized n-3 polyunsaturated fatty acids in chronic hepatitis C: correlation with hepatic steatosis. Journal of
gastroenterology 40, 617–624, doi: 10.1007/s00535-005-1596-x (2005). Article CAS PubMed Google Scholar * Zambo, V. et al. Lipotoxicity in the liver. World journal of hepatology 5,
550–557, doi: 10.4254/wjh.v5.i10.550 (2013). Article PubMed PubMed Central Google Scholar * Hiendleder, S., Zakhartchenko, V. & Wolf, E. Mitochondria and the success of somatic cell
nuclear transfer cloning: from nuclear-mitochondrial interactions to mitochondrial complementation and mitochondrial DNA recombination. Reproduction, fertility, and development 17, 69–83
(2005). Article CAS PubMed Google Scholar * Takeda, K. et al. Comparison of liver mitochondrial proteins derived from newborn cloned calves and from cloned adult cattle by
two-dimensional differential gel electrophoresis. Molecular reproduction and development 78, 263–273, doi: 10.1002/mrd.21298 (2011). Article CAS PubMed Google Scholar * Park, J. et al.
Disruption of Mitochondrion-To-Nucleus Interaction in Deceased Cloned Piglets. PloS one 10, e0129378, doi: 10.1371/journal.pone.0129378 (2015). Article CAS PubMed PubMed Central Google
Scholar * Ross, J. M. et al. High brain lactate is a hallmark of aging and caused by a shift in the lactate dehydrogenase A/B ratio. Proceedings of the National Academy of Sciences of the
United States of America 107, 20087–20092, doi: 10.1073/pnas.1008189107 (2010). Article ADS PubMed PubMed Central Google Scholar * Wang, Y. P. et al. Regulation of G6PD acetylation by
SIRT2 and KAT9 modulates NADPH homeostasis and cell survival during oxidative stress. The EMBO journal 33, 1304–1320, doi: 10.1002/embj.201387224 (2014). Article CAS PubMed PubMed Central
Google Scholar * Wu, L. E., Gomes, A. P. & Sinclair, D. A. Geroncogenesis: metabolic changes during aging as a driver of tumorigenesis. Cancer cell 25, 12–19, doi:
10.1016/j.ccr.2013.12.005 (2014). Article CAS PubMed PubMed Central Google Scholar * Perl, A., Hanczko, R., Telarico, T., Oaks, Z. & Landas, S. Oxidative stress, inflammation and
carcinogenesis are controlled through the pentose phosphate pathway by transaldolase. Trends in molecular medicine 17, 395–403, doi: 10.1016/j.molmed.2011.01.014 (2011). Article CAS PubMed
PubMed Central Google Scholar * Hocquette, J. F. & Bauchart, D. Intestinal absorption, blood transport and hepatic and muscle metabolism of fatty acids in preruminant and ruminant
animals. Reproduction, nutrition, development 39, 27–48 (1999). Article CAS PubMed Google Scholar * Laloë, D. et al. In 31st congress of european embryo transfer association (ed. Brazil
Brazilian College of Animal Reproduction) 818 (Animal Reproduction) (2015). * Betsha, S. et al. Transcriptome profile of bovine elongated conceptus obtained from SCNT and IVP pregnancies.
Molecular reproduction and development 80, 315–333, doi: 10.1002/mrd.22165 (2013). Article CAS PubMed Google Scholar * Bernard, L., Richard, C., Gelin, V., Leroux, C. & Heyman, Y.
Milk fatty acid composition and mammary lipogenic genes expression in bovine cloned and control cattle. Livestock Science 176, 188–195 (2015). Article Google Scholar * Scorletti, E. &
Byrne, C. D. Omega-3 fatty acids, hepatic lipid metabolism, and nonalcoholic fatty liver disease. Annual review of nutrition 33, 231–248, doi: 10.1146/annurev-nutr-071812-161230 (2013).
Article CAS PubMed Google Scholar * Martinez-Clemente, M. et al. Disruption of the 12/15-lipoxygenase gene (Alox15) protects hyperlipidemic mice from nonalcoholic fatty liver disease.
Hepatology 52, 1980–1991, doi: 10.1002/hep.23928 (2010). Article CAS PubMed Google Scholar * Rioux, V., Pedrono, F. & Legrand, P. Regulation of mammalian desaturases by myristic
acid: N-terminal myristoylation and other modulations. Biochimica et biophysica acta 1811, 1–8, doi: 10.1016/j.bbalip.2010.09.005 (2011). Article CAS PubMed Google Scholar * Teng, Y. B.
et al. Efficient demyristoylase activity of SIRT2 revealed by kinetic and structural studies. Scientific reports 5, 8529, doi: 10.1038/srep08529 (2015). Article CAS PubMed PubMed Central
Google Scholar * Liu, H. et al. Wnt signaling regulates hepatic metabolism. Science signaling 4, ra6, doi: 10.1126/scisignal.2001249 (2011). Article ADS CAS PubMed PubMed Central
Google Scholar * Grant, S. F. et al. Variant of transcription factor 7-like 2 (TCF7L2) gene confers risk of type 2 diabetes. Nature genetics 38, 320–323, doi: 10.1038/ng1732 (2006). Article
ADS CAS PubMed Google Scholar * Dayeh, T. et al. Genome-wide DNA methylation analysis of human pancreatic islets from type 2 diabetic and non-diabetic donors identifies candidate genes
that influence insulin secretion. PLoS genetics 10, e1004160, doi: 10.1371/journal.pgen.1004160 (2014). Article CAS PubMed PubMed Central Google Scholar * Musso, G. et al.
Transcription factor 7-like 2 polymorphism modulates glucose and lipid homeostasis, adipokine profile, and hepatocyte apoptosis in NASH. Hepatology 49, 426–435, doi: 10.1002/hep.22659
(2009). Article CAS PubMed Google Scholar * Ip, W., Shao, W., Chiang, Y. T. & Jin, T. The Wnt signaling pathway effector TCF7L2 is upregulated by insulin and represses hepatic
gluconeogenesis. American journal of physiology. Endocrinology and metabolism 303, E1166–1176, doi: 10.1152/ajpendo.00249.2012 (2012). Article CAS PubMed PubMed Central Google Scholar *
Gougelet, A. et al. T-cell factor 4 and beta-catenin chromatin occupancies pattern zonal liver metabolism in mice. Hepatology 59, 2344–2357, doi: 10.1002/hep.26924 (2014). Article CAS
PubMed Google Scholar * Schmidt, M., Sangild, P. T., Blum, J. W., Andersen, J. B. & Greve, T. Combined ACTH and glucocorticoid treatment improves survival and organ maturation in
premature newborn calves. Theriogenology 61, 1729–1744, doi: 10.1016/j.theriogenology.2003.10.002 (2004). Article CAS PubMed Google Scholar * Shaikh, S. R., Kinnun, J. J., Leng, X.,
Williams, J. A. & Wassall, S. R. How polyunsaturated fatty acids modify molecular organization in membranes: insight from NMR studies of model systems. Biochimica et biophysica acta
1848, 211–219, doi: 10.1016/j.bbamem.2014.04.020 (2015). Article CAS PubMed Google Scholar * Chavatte-Palmer, P. et al. Clinical, hormonal, and hematologic characteristics of bovine
calves derived from nuclei from somatic cells. Biology of reproduction 66, 1596–1603 (2002). Article CAS PubMed Google Scholar * Hinds, T. D. Jr. et al. Protein phosphatase 5 mediates
lipid metabolism through reciprocal control of glucocorticoid receptor and peroxisome proliferator-activated receptor-gamma (PPARgamma). The Journal of biological chemistry 286, 42911–42922,
doi: 10.1074/jbc.M111.311662 (2011). Article CAS PubMed PubMed Central Google Scholar * Hirayama, H. et al. Prepartum maternal plasma glucose concentrations and placental glucose
transporter mRNA expression in cows carrying somatic cell clone fetuses. The Journal of reproduction and development 57, 57–61 (2011). Article CAS PubMed Google Scholar * Rousseau, D.,
Helies-Toussaint, C., Moreau, D., Raederstorff, D. & Grynberg, A. Dietary n-3 PUFAs affect the blood pressure rise and cardiac impairments in a hyperinsulinemia rat model _in vivo_.
American journal of physiology. Heart and circulatory physiology 285, H1294–1302, doi: 10.1152/ajpheart.00651.2002 (2003). Article CAS PubMed Google Scholar * Kiefer, H. Genome-Wide
Analysis of Methylation in Bovine Clones by Methylated DNA Immunoprecipitation (MeDIP). Methods in molecular biology 1222, 267–280, doi: 10.1007/978-1-4939-1594-1_20 (2015). Article CAS
PubMed Google Scholar * Mi, H., Muruganujan, A. & Thomas, P. D. PANTHER in 2013: modeling the evolution of gene function, and other gene attributes, in the context of phylogenetic
trees. Nucleic acids research 41, D377–386, doi: 10.1093/nar/gks1118 (2013). Article CAS PubMed Google Scholar * Guillomot, M. et al. Spatial and temporal changes of Decorin, Type I
collagen and Fibronectin expression in normal and clone bovine placenta. Placenta 35, 737–747, doi: 10.1016/j.placenta.2014.06.366 (2014). Article CAS PubMed Google Scholar *
Vandesompele, J. et al. Accurate normalization of real-time quantitative RT-PCR data by geometric averaging of multiple internal control genes. Genome biology 3, RESEARCH0034 (2002). *
Goossens, K. et al. Selection of reference genes for quantitative real-time PCR in bovine preimplantation embryos. BMC developmental biology 5, 27, doi: 10.1186/1471-213X-5-27 (2005).
Article CAS PubMed PubMed Central Google Scholar Download references ACKNOWLEDGEMENTS This work was supported by grants from the French National Research Agency (ANR-09-GENM-012-01),
APIS-GENE (13000394) and INRA’s Animal Physiology and Livestock Systems Division (ACI PHASE 2010). We thank Michel Guillomot, Véronique Duranthon, Claudine Junien, Sophie Calderari and
Delphine Dubé for helpful discussions, Nicolas Le Cleac’h for assistance with ultrasound imaging, Valérie Gélin and Alexandre Neveux for their help with the animals, Marion Souchal for her
assistance with the pyrosequencing of _TCF7L2_ and François Casas for his critical reading of the manuscript. IPS2 benefits from the support of the LabEx Saclay Plant Sciences-SPS
(ANR-10-LABX-0040-SPS). AUTHOR INFORMATION Author notes * Present address: IRHS, INRA, AGROCAMPUS-Ouest, Université d’Angers, SFR 4207 QUASAV, 42 rue Georges Morel, 49071 Beaucouzé cedex,
France. * Present address: ALLICE, lieu-dit Le Perroi, Nouzilly, France. AUTHORS AND AFFILIATIONS * UMR BDR, INRA, ENVA, Université Paris Saclay, Jouy en Josas, 78350, France Hélène Kiefer,
Luc Jouneau, Évelyne Campion, Delphine Rousseau-Ralliard, Audrey Prézelin, Pascale Chavatte-Palmer, Yvan Heyman, Christophe Richard, Daniel Le Bourhis, Jean-Paul Renard & Hélène Jammes *
INRA, UMR0703 APEX, Oniris, Nantes, France Thibaut Larcher & Mireille Ledevin * UMR MIA-Paris, AgroParisTech, INRA, Université Paris-Saclay, Paris, 75005, France Marie-Laure
Martin-Magniette * Institute of Plant Sciences Paris Saclay IPS2, CNRS, INRA, Université Paris-Sud, Université Evry, Université Paris-Saclay, Orsay, 91405, France Marie-Laure
Martin-Magniette & Sandrine Balzergue * Institute of Plant Sciences Paris-Saclay IPS2, Paris Diderot, Sorbonne Paris-Cité, Bâtiment 630, Orsay, 91405, France Marie-Laure Martin-Magniette
* INRA, UE1298, Unité Commune d’Expérimentation Animale, Leudeville, France Christophe Richard Authors * Hélène Kiefer View author publications You can also search for this author inPubMed
Google Scholar * Luc Jouneau View author publications You can also search for this author inPubMed Google Scholar * Évelyne Campion View author publications You can also search for this
author inPubMed Google Scholar * Delphine Rousseau-Ralliard View author publications You can also search for this author inPubMed Google Scholar * Thibaut Larcher View author publications
You can also search for this author inPubMed Google Scholar * Marie-Laure Martin-Magniette View author publications You can also search for this author inPubMed Google Scholar * Sandrine
Balzergue View author publications You can also search for this author inPubMed Google Scholar * Mireille Ledevin View author publications You can also search for this author inPubMed Google
Scholar * Audrey Prézelin View author publications You can also search for this author inPubMed Google Scholar * Pascale Chavatte-Palmer View author publications You can also search for
this author inPubMed Google Scholar * Yvan Heyman View author publications You can also search for this author inPubMed Google Scholar * Christophe Richard View author publications You can
also search for this author inPubMed Google Scholar * Daniel Le Bourhis View author publications You can also search for this author inPubMed Google Scholar * Jean-Paul Renard View author
publications You can also search for this author inPubMed Google Scholar * Hélène Jammes View author publications You can also search for this author inPubMed Google Scholar CONTRIBUTIONS
H.K., J.P.R. and H.J. designed and coordinated the study. H.K. wrote the manuscript. H.K., E.C., D.R.R., T.L., S.B., M.L., A.P., P.C.P., Y.H., C.R. and D.L.B. collected the samples and
performed the experiments. L.J. and M.L.M.M. performed bioinformatics analyses. All authors read and approved the final manuscript. ETHICS DECLARATIONS COMPETING INTERESTS The authors
declare no competing financial interests. ELECTRONIC SUPPLEMENTARY MATERIAL SUPPLEMENTARY INFORMATION SUPPLEMENTARY DATASET 1 SUPPLEMENTARY DATASET 2 SUPPLEMENTARY DATASET 3 SUPPLEMENTARY
DATASET 4 RIGHTS AND PERMISSIONS This work is licensed under a Creative Commons Attribution 4.0 International License. The images or other third party material in this article are included
in the article’s Creative Commons license, unless indicated otherwise in the credit line; if the material is not included under the Creative Commons license, users will need to obtain
permission from the license holder to reproduce the material. To view a copy of this license, visit http://creativecommons.org/licenses/by/4.0/ Reprints and permissions ABOUT THIS ARTICLE
CITE THIS ARTICLE Kiefer, H., Jouneau, L., Campion, É. _et al._ Altered DNA methylation associated with an abnormal liver phenotype in a cattle model with a high incidence of perinatal
pathologies. _Sci Rep_ 6, 38869 (2016). https://doi.org/10.1038/srep38869 Download citation * Received: 08 June 2016 * Accepted: 14 November 2016 * Published: 13 December 2016 * DOI:
https://doi.org/10.1038/srep38869 SHARE THIS ARTICLE Anyone you share the following link with will be able to read this content: Get shareable link Sorry, a shareable link is not currently
available for this article. Copy to clipboard Provided by the Springer Nature SharedIt content-sharing initiative